- Open access
- Published: 02 January 2020

Development of therapeutic antibodies for the treatment of diseases
- Ruei-Min Lu 1 ,
- Yu-Chyi Hwang 1 ,
- I-Ju Liu 1 na1 ,
- Chi-Chiu Lee 1 na1 ,
- Han-Zen Tsai 1 na1 ,
- Hsin-Jung Li 1 &
- Han-Chung Wu ORCID: orcid.org/0000-0002-5185-1169 1 , 2
Journal of Biomedical Science volume 27 , Article number: 1 ( 2020 ) Cite this article
414k Accesses
1297 Citations
287 Altmetric
Metrics details
It has been more than three decades since the first monoclonal antibody was approved by the United States Food and Drug Administration (US FDA) in 1986, and during this time, antibody engineering has dramatically evolved. Current antibody drugs have increasingly fewer adverse effects due to their high specificity. As a result, therapeutic antibodies have become the predominant class of new drugs developed in recent years. Over the past five years, antibodies have become the best-selling drugs in the pharmaceutical market, and in 2018, eight of the top ten bestselling drugs worldwide were biologics. The global therapeutic monoclonal antibody market was valued at approximately US$115.2 billion in 2018 and is expected to generate revenue of $150 billion by the end of 2019 and $300 billion by 2025. Thus, the market for therapeutic antibody drugs has experienced explosive growth as new drugs have been approved for treating various human diseases, including many cancers, autoimmune, metabolic and infectious diseases. As of December 2019, 79 therapeutic mAbs have been approved by the US FDA, but there is still significant growth potential. This review summarizes the latest market trends and outlines the preeminent antibody engineering technologies used in the development of therapeutic antibody drugs, such as humanization of monoclonal antibodies, phage display, the human antibody mouse, single B cell antibody technology, and affinity maturation. Finally, future applications and perspectives are also discussed.
Monoclonal antibodies (mAbs) are produced by B cells and specifically target antigens. The hybridoma technique introduced by Köhler and Milstein in 1975 [ 1 ] has made it possible to obtain pure mAbs in large amounts, greatly enhancing the basic research and potential for their clinical use. Other scientific and technological advances have also enabled the successful translation of mAbs to the clinic. Around the world, at least 570 therapeutic mAbs have been studied in clinical trials by commercial companies [ 2 ], and 79 therapeutic mAbs have been approved by the United States Food and Drug Administration (US FDA) and are currently on the market [ 3 ], including 30 mAbs for the treatment of cancer (Table 1 ).
The increasing importance of therapeutic mAbs is apparent (Fig. 1 ), as mAbs have become the predominant treatment modality for various diseases over the past 25 years. During this time, major technological advances have made the discovery and development of mAb therapies quicker and more efficient. Since 2008, 48 new mAbs have been approved, contributing to a total global market of 61 mAbs in clinical use at the end of 2017, according to the US FDA. Strikingly, a total of 18 new antibodies were granted approval by the US FDA from 2018 to 2019 – this number was tallied from information contained on various websites, including the antibody society [ 3 ], the database of therapeutic antibodies [ 4 ], and company pipelines and press releases. A list of antibody-based drugs approved by the US FDA is shown in Table 1 .
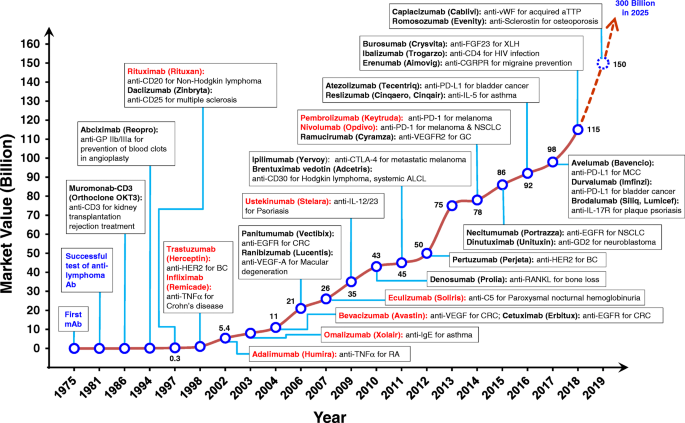
Timeline from 1975 showing the successful development of therapeutic antibodies and their applications. Many biotech companies that promised antibodies as anticancer “magic bullets” were launched from 1981 to 1986. The height of the line and numerical annotations represent the estimated market value of mAb therapeutics in each indicated year (shown as billions of US dollars). Antibodies colored in red represent the top 10 best-selling antibody drugs in 2018. Ab, antibody; ALCL, systematic anaplastic large-cell lymphoma; aTTP, acquired thrombotic thrombocytopenic purpura; BC, breast cancer; CD, cluster of differentiation; CGRP, calcitonin gene-related peptide; CGRPR, calcitonin gene-related peptide receptor; CRC, colorectal cancer; CTLA-4, cytotoxic T-lymphocyte-associated protein 4; EGFR, epidermal growth factor receptor; FGF, fibroblast growth factor; GC, gastric cancer; GD2, disialoganglioside G D2 ; HER2, human epidermal growth factor receptor 2; IgE, immunoglobulin E; IL, interleukin; IL-17R, interleukin-17 receptor; mAb, monoclonal antibody; MCC, merkel-cell carcinoma; NSCLC, non-small cell lung cancer; PD-1, programmed cell death protein 1; PD-L1, programmed death-ligand 1; TNFα, tumor necrosis factor α; RA, rheumatoid arthritis; RANKL, receptor activator of nuclear factor kappa-B ligand; VEGF-A, vascular endothelial growth factor A; VEGFR2, vascular endothelial growth factor receptor 2; vWF, von Willebrand factor; XLH, X-linked hypophosphatemia
The first therapeutic mAb, muromonab-CD3 (Orthoclone OKT3), was approved by the US FDA in 1986 [ 5 ] and comprises a murine mAb against T cell-expressed CD3 that functions as an immunosuppressant for the treatment of acute transplant rejection. The marketing end date of muromonab-CD3 is on July 30th, 2011 (Table 1 ). To overcome problems of decreased immunogenic potential and efficacy, while making possible the therapeutic use of antibodies for an extended duration, researchers developed techniques to transform rodent antibodies into structures more similar to human antibodies, without loss of binding properties. The first chimeric antibody, anti-GPIIb/IIIa antigen-binding fragment (Fab) (abciximab), was approved in 1994 by the US FDA for inhibition of platelet aggregation in cardiovascular diseases (Fig. 1 ). The drug was developed by combining sequences of the murine variable domain with human constant region domain (Fig. 2b ) [ 6 , 7 ]. Then the first mAb with an oncologic indication, rituximab, a chimeric anti-CD20 IgG1 approved for non-Hodgkin’s lymphoma in 1997 by US FDA (Fig. 1 ) [ 8 , 9 ].
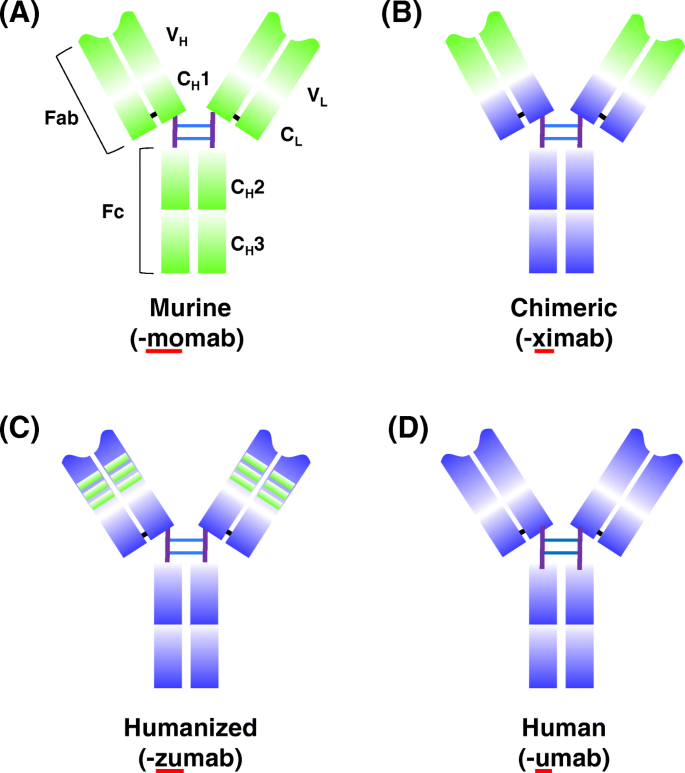
Schematic overview of antibody humanization from murine antibodies (green domains) to fully human antibodies (orange domains) and associated suffixes. a The murine monoclonal antibody. b The chimeric monoclonal antibody: variable regions are of murine origin, and the rest of the chains are of human origin. c Humanized monoclonal antibody: only includes the hypervariable segments of murine origin. d Human monoclonal. C H : domains of the constant region of the heavy chain; C L : constant domain of the light chain; Fab and Fc: fragments resulting from proteolysis; V H : variable domain of the heavy chain; V L : variable domain of the light chain
One exceptional advance that accelerated the approval of therapeutic mAbs was the generation of humanized antibodies by the complementary-determining region (CDR) grafting technique [ 10 ]. In CDR grafting, non-human antibody CDR sequences are transplanted into a human framework sequence in order to maintain target specificity [ 10 ] (Fig. 2c ). The first humanized mAb approved by the US FDA in 1997 was the anti-IL-2 receptor, daclizumab, for the prevention of transplant rejection (Fig. 1 ) [ 11 ]. The humanization of antibodies made it possible to clinically apply a new class of biologics directed against diseases that require long-term treatment, such as cancer and autoimmune diseases [ 12 ].
Based on the success of humanized mAbs in the clinic, a key discovery technology to obtain fully human mAbs (Fig. 2d ) was developed in 1990 by Sir Gregory P. Winter [ 10 , 13 ]. This technique was based on phage display, wherein diverse exogenous genes are incorporated into filamentous bacteriophages to compose a library. The library proteins are then presented on the phage surface as fusions with a phage coat protein, allowing the selection of specific binders and affinity characteristics. The phage display technique was first introduced by George P. Smith [ 14 ] and comprises a powerful method for the rapid identification of peptides or antibody fragments, such as single chain fragment variable (scFv) or Fab, that bind a variety of target molecules (proteins, cell-surface glycans and receptors) [ 15 ] (Fig. 3b ). The Nobel Prize in Chemistry 2018 was awarded to George P. Smith and Sir Gregory P. Winter. George Smith developed phage-displayed peptides, which can be used to evolve new proteins [ 14 ]. Gregory P. Winter was able to apply the phage-displayed antibody library to the discovery and isolation of antibodies [ 13 ]. Phage display technology has also been used for antibody maturation by site-directed mutagenesis of CDR and affinity selection. Based on these techniques, the first fully human therapeutic antibody, adalimumab (Humira), an anti-tumor necrosis factor α (TNFα) human antibody [ 16 ], was approved in 2002 by the US FDA for rheumatoid arthritis (Fig. 1 ). Until now, nine human antibody drugs generated by phage display have been approved by the US FDA (Table 5 ).
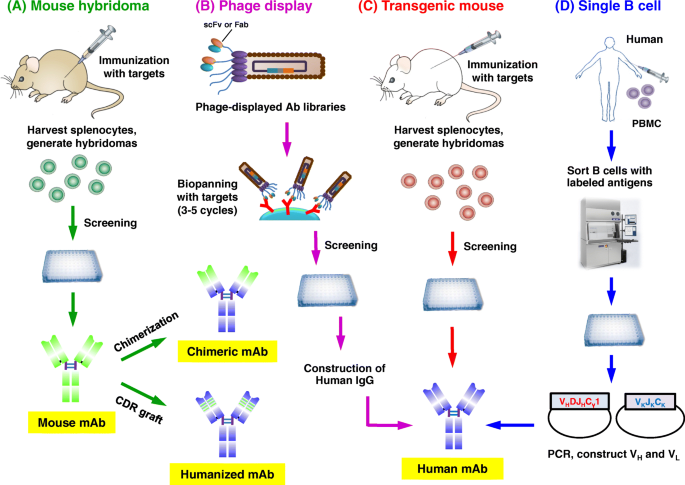
Approaches for the development of therapeutic antibodies. a The traditional mouse hybridoma technique starts by immunization of mice with desired antigens to trigger an immune response. Harvested splenocytes are fused with myeloma cells to produce hybridoma cells that persistently secrete antibodies. After the screening, selected leads are used to generate chimeric or humanized antibodies. b Phage display. A human phage-displayed human antibody library is used to select antigens of interest. After 3–5 rounds of biopanning, immuno-positive phage clones are screened by ELISA; then DNA sequences are analyzed to construct and express human IgGs. c Transgenic mouse. Similar to the mouse hybridoma technique or single B cell methods. d The single B cell technique. From infected or vaccinated donors, PBMCs are prepared for isolation of suitable B cells by flow cytometry. Following the RT-PCR, V H and V L information of each B cell informs the generation of human mAbs
Transgenic animals represent another technology for obtaining fully human mAbs (Fig. 3c ). This technology was introduced in 1994 by the publication of two transgenic mouse lines, the HuMabMouse [ 35 ] and the XenoMouse [ 36 ]. The lines were genetically modified such that human immunoglobulin (Ig) genes were inserted into the genome, replacing the endogenous Ig genes and making these animals capable of synthesizing fully human antibodies upon immunization [ 35 , 37 ]. The first human antibody generated in a transgenic mouse to anti-epidermal growth factor receptor (EGFR), panitumumab, was approved by the US FDA in 2006 (Fig. 1 ) [ 38 , 39 ]. The number of fully human antibodies made from transgenic mice has increased rapidly, with the number of approved drugs currently at 19 (Table 5 ). Depending on the immunization protocol, high-affinity human antibodies can be obtained through further selection of hybridoma clones generated from immunized transgenic mice. Using a theoretically similar approach, the generation of neutralizing human antibodies from human B cells has also yielded promising results for infectious disease therapeutics.
The recent development of bispecific antibodies offers attractive new opportunities for the design of novel protein therapeutics. A bispecific antibody can be generated by utilizing protein engineering techniques to link two antigen binding domains (such as Fabs or scFvs), allowing a single antibody to simultaneously bind different antigens. Thus, bispecific antibodies may be engineered to exhibit novel functions, which do not exist in mixtures of the two parental antibodies. Most bispecific antibodies are designed to recruit cytotoxic effector cells of the immune system to target pathogenic cells [ 40 ]. The first approved bispecific antibody was catumaxomab in Europe in 2009 [ 41 ]. Catumaxomab targets CD3 and EpCAM to treat solid tumors in patients with malignant ascites. However, this drug was withdrawn from the market in 2017 for commercial reasons. Currently, two bispecific antibodies have obtained US FDA approval and are on the market. First, blinatumomab is a bispecific T-cell engager (BiTE) that targets CD3 and CD19 for treatment of B-cell precursor acute lymphoblastic leukemia (ALL) [ 42 ]. Second, emicizumab is a full-size bispecific IgG with natural architecture, which binds to activated coagulation factors IX and X for the treatment of haemophilia A [ 43 ]. To date, there are more than 85 bispecific antibodies in clinical trials, about 86% of which are under evaluation as cancer therapies [ 40 ]. The concepts and platforms driving the development of bispecific antibodies continue to advance rapidly, creating many new opportunities to make major therapeutic breakthroughs.
While mAbs are routinely used in biochemistry, molecular and cellular biology, and medical research, perhaps the most beneficial application is their use as therapeutic drugs for the treatment of human diseases, such as cancer, asthma, arthritis, psoriasis, Crohn’s disease, transplant rejection, migraine headaches and infectious diseases (Table 1 ). Important advances in antibody engineering made over the past decade have enhanced the safety and efficacy of the therapeutic antibodies. These developments, along with a greater understanding of the immunomodulatory properties of antibodies, have paved the way for the next generation of new and improved antibody-based drugs for the treatment of human diseases.
Clinical applications and market for therapeutic antibodies
Therapeutic antibodies currently approved as disease treatments.
The mAb market enjoys a healthy pipeline and is expected to grow at an increasing pace, with a current valuation of $115.2 billion in 2018 [ 44 ]. Despite this high growth potential, new companies are unlikely to take over large shares of the market, which is currently dominated by seven companies: Genentech (30.8%), Abbvie (20.0%), Johnson & Johnson (13.6%), Bristol-Myers Squibb (6.5%), Merck Sharp & Dohme (5.6%), Novartis (5.5%), Amgen (4.9%), with other companies comprising the remaining 13% [ 44 ].
Many mAbs products achieved annual sales of over US$3 billion in 2018 (Fig. 1 ), while six (adalimumab, nivolumab, pembrolizumab, trastuzumab, bevacizumab, rituximab) had sales of more than $6 billion (Table 2 ). Adalimumab (Humira) had the highest sales figure ever recorded for a biopharmaceutical product, nearly $19.9 billion. The top ten selling mAb products in 2018 are listed in Table 2 . Top-selling mAb drugs were ranked based on sales or revenue reported by biological or pharmacological companies in press announcements, conference calls, annual reports or investor materials throughout 2018. For each drug, the name, sponsors, disease indications, and 2018 sales are shown.
mAbs are increasingly used for a broad range of targets; oncology, immunology, and hematology remain the most prevalent medical applications [ 45 ]. Most mAbs have multiple disease indications and at least one that is cancer-related (lymphoma, myeloma, melanoma, glioblastoma, neuroblastoma, sarcoma, colorectal, lung, breast, ovarian, head and neck cancers). As such, oncological diseases are the medical specialty most accessible to mAb treatments [ 45 ]. Moreover, the number of target proteins known to function as either stimulatory or inhibitory checkpoints of the immune system has dramatically expanded, and numerous antibody therapeutics targeting programmed cell death protein 1 (PD-1, cemiplimab, nivolumab, pembrolizumab), its ligand programmed death-ligand 1 (PD-L1, durvalumab, avelumab, atezolizumab) or cytotoxic T-lymphocyte–associated antigen 4 (CTLA-4, ipilimumab) have been granted marketing approvals [ 46 ].
Adalimumab (Humira) was the world’s best-selling drug in 2018. Adalimumab is a subcutaneously administered biological disease modifier used for the treatment of rheumatoid arthritis and other TNFα-mediated chronic debilitating diseases. It was originally launched by Abbvie in the United States after gaining approval from the US FDA in 2002. It has been shown that Adalimumab reduces the signs and symptoms of moderate to severe rheumatoid arthritis in adults, and it is also used to treat psoriatic arthritis, ankylosing spondylitis, Crohn's disease, ulcerative colitis, psoriasis, hidradenitis suppurativa, uveitis, and juvenile idiopathic arthritis [ 47 , 48 ]. It may be used alone or in combination with disease-modifying anti-rheumatic drugs [ 49 ].
Immune checkpoints are important for maintaining self-tolerance and tempering physiologic immune responses in peripheral tissues. Therefore, the molecules underlying checkpoints have recently drawn considerable interest in cancer immunotherapy [ 50 ]. Both nivolumab (Opdivo) and pembrolizumab (Keytruda) are anti-PD-1 mAbs and were the second and third best-selling mAb drugs in 2018 (Table 2 ). Nivolumab is a human antibody, which blocks a signal that normally prevents activated T cells from attacking cancer cells. The target for nivolumab is the PD-1 receptor, and the antibody blocks the interaction of PD-1 with its ligands, PD-L1 and PD-L2, releasing PD-1 pathway-mediated immune inhibition [ 51 , 52 ]. Pembrolizumab is a humanized antibody used in cancer immunotherapy to treat melanoma, lung cancer, head and neck cancer, Hodgkin’s lymphoma, and stomach cancer [ 53 , 54 , 55 ]. Pembrolizumab is a first-line treatment for NSCLC if cancer cells overexpresse PD-L1 and have no mutations in EGFR or in anaplastic lymphoma kinase [ 56 , 57 ]. Large randomized clinical trials indicated that NSCLC patients treated with nivolumab and pembrolizumab (both approved by the US FDA in 2014) showed increased overall survival compared with docetaxel, the standard second-line treatment [ 58 ].
A total of 12 new mAbs were approved in the US during 2018. The majority of these products were approved for non-cancer indications, perhaps reflecting the higher approval success rate for antibodies as treatments for other diseases. Three antibodies (erenumab, galcanezumab, and fremaezumab) were approved for migraine prevention, and one (Ibalizumab) is used for human immunodeficiency virus (HIV) infection. The three migraine-preventing drugs, Erenumab (Aimovig), galcanezumab (Emgality), and fremaezumab (Ajovy), are mAbs that block the activity of calcitonin gene-related peptide (CGRP) receptor in migraine etiology [ 59 ]. CGRP acts through a heteromeric receptor, which is composed of a G protein-coupled receptor(calcitonin receptor-like receptor: CALCRL) and receptor activity-modifying protein 1 (RAMP1) [ 60 , 61 ]. Both galcanezumab and fremaezumab bind to CGRP and block its binding to the receptor. However, erenumab is the only one of the three antibodies to target the extracellular domains of human G protein-coupled receptors CALCRL and RAMP1,interfering with the CGRP binding pocket [ 62 ].
Many mAbs are under development for treatment of infectious diseases, currently only four have been approved by the US FDA: raxibacumab and obiltoxaximab for treatment of inhalational anthrax [ 63 ], palivizumab for prevention of respiratory syncytial virus in high-risk infants [ 64 ], and ibalizumab for treatment of HIV infection patients [ 65 ]. Ibalizumab (Trogarzo) is a humanized IgG4 mAb that is used as a CD4 domain 2-directed post-attachment HIV-1 inhibitor. The US FDA approved ibalizumab for adult patients infected with HIV who were previously treated and are resistant to currently available therapies.
Therapeutic antibodies currently in clinical trials
Companies are currently sponsoring clinical studies for more than 570 mAbs. Of these, approximately 90% are early-stage studies designed to assess safety (Phase I) or safety and preliminary efficacy (Phase I/II or Phase II) in patient populations. Most of the mAbs in Phase I (~ 70%) are for cancer treatment, and the proportions of mAbs intended to treat cancer are similar for those currently in Phase II and late-stage clinical studies (pivotal Phase II, Phase II/III or Phase III) [ 2 ].
Twenty-nine novel antibody therapeutics were in late-stage clinical studies for non-cancer indications in 2018. Among the trials for these mAbs, no single therapeutic area predominated, but 40% were for immune-mediated disorders, which comprised the largest group. From this group of potential treatments, leronlimab and brolucizumab entered regulatory review by the end of 2018, and five mAbs (eptinezumab, teprotumumab, crizanlizumab, satralizumab, and tanezumab) may enter regulatory review in 2019. In comparison, there were 33 novel antibody therapeutics in late-stage clinical studies for cancer indications in 2018. Antibody therapeutics for solid tumors clearly predominated, with less than 20% of the candidates intended solely for hematological malignancies. Five mAbs (isatuximab, spartalizumab, tafasitamab, dostarlimab, and ublituximab) license applications were submitted to the US FDA in 2019 [ 2 ].
Isatuximab is an anti-CD38 IgG1 chimeric mAb under evaluation as a treatment for patients with multiple myeloma (MM). Combinations of isatuximab and different chemotherapies are being tested in three Phase III studies (ICARIA, IKEMA, and IMROZ) on MM patients. The ICARIA study (NCT02990338) is evaluating the effects of isatuximab in combination with pomalidomide and dexamethasone compared to chemotherapy only in patients with refractory or relapsed MM. Pivotal Phase III ICARIA-MM trial results demonstrated that isatuximab combination therapy showed statistically significant improvements compared to pomalidomide and dexamethasone alone in patients with relapsed or refractory MM in 2019. The US FDA has accepted for review the biologics license application for isatuximab for the treatment relapsed or refractory MM patients. The target action date for the FDA decision is April 2020 [ 66 ]. The IKEMA (NCT03275285) and IMROZ (NCT03319667) studies are evaluating the isatuximab with other chemotherapeautic combinations in MM patients [ 67 ].
Spartalizumab is a humanized IgG4 mAb that binds PD-1 with sub-nanomolar affinity and blocks its interaction with PD-L1/PD-L2, preventing PD-1-mediated inhibitory signaling and leading to T-cell activation. Clinical study of Spartalizumab is underway with a randomized, double-blind, placebo-controlled Phase III COMBI-i study (NCT02967692), which is evaluating the safety and efficacy of dabrafenib and trametinib in combination with spartalizumab compared to matching placebo in previously untreated patients with BRAF V600-mutant unresectable or metastatic melanoma. The primary endpoints of the study are an assessment of dose-limiting toxicities, changes in PD-L1 levels and CD8+ cells in the tumor microenvironment, and progression-free survival. Key secondary endpoints are overall survival, overall response rate and duration of response. The estimated primary completion date of the study is September 2019 [ 68 ].
Dostarlimab is an anti-PD-1 mAb that may be useful as a treatment for several types of cancers. GlaxoSmithKline announced results from a Phase I dose escalation and cohort expansion study (GARNET; NCT02715284) in 2018, which is expected to support a biologics license application submission to the US FDA in 2019. Dostarlimab is being assessed in patients with advanced solid tumors who have limited available treatment options in the GARNET study. The drug is administered at a dose of 500 mg every 3 weeks for the first 4 cycles, and 1000 mg every 6 weeks thereafter in four patient cohorts: microsatellite instability high (MSI-H) endometrial cancer, MSI-H non-endometrial cancer, microsatellite-stable endometrial cancer, and non-small cell lung cancer. Dostarlimab is also being evaluated in another Phase III study (NCT03602859), which is comparing platinum-based therapy with dostarlimab and niraparib versus standard of care platinum-based therapy as first-line treatment of Stage III or IV non-mucinous epithelial ovarian cancer [ 69 ].
Ublituximab is a glyco-engineered anti-CD20 antibody currently under clinical investigation in five late-stage clinical studies for different cancers (chronic lymphocytic leukemia, CLL, non-Hodgkin’s lymphoma) and non-cancer (multiple sclerosis) indications. Three Phase III studies are exploring the efficacy of ublituximab in combination with other anti-cancer agents. Among these studies, the UNITY-CLL Phase III study (NCT02612311) is evaluating the combination of ublituximab and TGR-1202, a PI3K delta inhibitor, compared to anti-CD20 obinutuzumab plus chlorambucil in untreated and previously treated CLL patients. Two other Phase III studies (ULTIMATE 1, NCT03277261 and ULTIMATE 2, NCT03277248) are evaluating the efficacy and safety of ublituximab compared to teriflunomide in 440 patients with relapsing multiple sclerosis [ 70 ].
Methodologies for developing therapeutic antibodies
Human, humanized, chimeric, and murine antibodies respectively account for 51, 34.7, 12.5, and 2.8% of all mAbs in clinical use, making human and humanized mAbs the dominant modalities in the field of therapeutic antibodies. In the next section, we first introduce techniques for antibody humanization. Then, we describe three technical platforms related to the generation of fully human antibodies, including phage display, transgenic mice and single B cell antibody isolation (Fig. 3 ). Last, we describe the use of an affinity maturation method to optimize antibody binding activity.
Humanization of mAbs
Due to the availability, low cost and quick production time for mouse mAbs, humanization of mouse mAbs has been implemented on a large scale. Non-humanized murine mAbs have many disadvantages as treatments. For example, patients treated with mouse mAbs will produce a rapid human anti-mouse antibody (HAMA) response. HAMAs will not only hasten the clearance of mouse mAbs but may also produce undesirable allergic reactions and tumor penetration. Moreover, the ability of patients to initiate antibody-dependent cellular cytotoxicity (ADCC) in response to murine fragment crystallizable region (Fc) is limited. On the other hand, humanized mAbs are able to effectively exert effector functions while decreasing the immunogenicity of murine antibodies.
Generation of humanized mAbs
Humanized mAbs, of which only the CDRs of the light and heavy chains are murine, entered clinical development for the first time in 1988 [ 71 , 72 ]. CDR grafting is one of the most popular techniques in the production of humanized mAbs and was originally developed by Gregory P. Winter in 1986 [ 9 ]. Using this technology, non-human CDR sequences are transplanted into human framework sequences, allowing the antibody to maintain the binding activity to the target antigen [ 9 ]. The first US FDA approved CDR-grafted humanized mAb occurred in 1997 for daclizumab, which binds the IL-2 receptor and is used to prevent transplant rejection [ 11 ]. Queen and collaborators [ 73 ] developed daclizumab not only using CDR grafting, but also using the human framework that is maximally homologous to the murine framework, in order to decrease the loss of antigen recognition. In some cases, certain amino acids in the murine framework are crucial to maintain antibody binding activity. These residues may cooperate with CDRs to present an antibody paratope or directly interact with antigens. Currently, these crucial framework residues can be identified by observing the structure of antibody-antigen complex by X-ray crystallography, cryo-electron microscopy and computer-aided protein homology modelling [ 74 ]. The positions of amino acids in the framework may then be considered for restore by ‘human back to mouse’ mutations in CDR-grafted humanized antibodies, thereby improving the affinity and stability of the final product. Currently, web servers are being developed by integrated bioinformatics and antibody structure databases for rendering humanization experiments [ 75 , 76 ]. They provide the tools for human template selection, grafting, back-mutation evaluation, and antibody modeling. However, if the binding activity of antibodies is still compromised, it should be further performed affinity maturation to improve this situation.
Multiple methods have been developed to quantify the humanness of the variable region of mAbs. Abhinandan and Martin designed a tool called “H-score” to assess the “degree of humanness” of antibody sequences, which calculates the mean sequence identity compared to a subset of human variable region sequences database [ 77 ]. A germinality index was defined subsequent to assist germline humanization of a macaque antibody [ 78 ]. G-score was derived from the H-score to improve classification of germline framework sequence [ 79 ]. T20 score analyzer was established under a large database of ~ 38,700 human antibody variable region sequences to clearly separate human sequences from mouse sequences and many other species as well [ 80 ]. It was used to reveal similarities between humanized antibodies and fully human antibodies. These humanness score tools are available online and allow assisting the generation of humanized antibody [ 80 ].
The use of humanized antibodies has helped greatly to improve clinical tolerance of mAb therapeutics. Such intricate control over antibody sequences has opened the door to engineering mAbs for a wide range of possible applications in medicine. Currently, half of all mAbs used to treat humans are chimeric or humanized (Fig. 2 , Table 1 ). One of the most well-known humanized antibodies is Trastuzumab (Herceptin), which was approved in 1998 and achieved annual sales of over $7 billion in 2018 (Table 2 ). Trastuzumab is used for the treatment of patients with human epidermal growth factor receptor 2 (HER2)-positive metastatic breast cancer and gastroesophageal junction adenocarcinoma [ 57 , 58 ].
Immunogenicity of antibody-based therapeutics
The use of mAbs in a clinical setting should have several essential biophysical properties, including high antigen binding activity, high stability, and low immunogenicity [ 81 ]. Antibody immunogenicity means the degree of the host immune system can recognize and react to these therapeutic agents. Anti-drug antibodies (ADA) induced by the immune system can be found while immunogenicity occurring in patients administered with antibody drugs. Anti-drug antibodies have the potential to neutralize therapeutic agents, which can reduce the efficacy of the drugs [ 82 ]. Importantly, anti-drug antibodies may further cause adverse effects ranging from skin rashes to systemic inflammatory responses in the patients, which can impact both safety and efficacy of the antibody drugs in clinic use [ 83 ]. Immunogenicity is influenced by several factors, such as drug dosage, administration strategy (route and combination), impurities contamination, aggregates arising from Ab/Ag binding complex, and structural features (sequence variation and glycosylation) [ 84 ].
Humanized antibodies harbor human sequence in constant regions and nearly all human sequence in Fv, of which only CDRs are murine grafted. Antibodies of more human-like usually allow them to be higher tolerant and lower immunogenic in a clinical setting. For example, Perpetua et al. showed a case to support this concept [ 85 ]. They compared a humanized anti-CD52 antibody with its parental murine version and demonstrated humanization offers a significant reduction in immunogenicity. However, humanized antibodies retain murine CDRs which could be regarded as foreign antigens by host immune systems and eventually arise immunogenicity. For example, ADA was detected in 0.5% of women with metastatic breast cancer, who were treated with Trastuzumab during their therapeutic courses [ 86 ]. Recently, an immunogenicity analysis result from clinical data showed the ADA rates were 7.1% (21/296) in the HER-2 positive breast cancer patients with treatment of Trastuzumab [ 87 ]. The variation of immunogenicity in the same antibody drug may be caused by many potential factors: the age, race, genetic background, other related diseases, and programs of drugs administration.
The CDRs and frameworks of fully human antibodies are derived for human immunoglobulin gene repertoires, thus which can theoretically bypass immunogenicity. However, several fully human antibodies have been reported to induce marked immune responses when administrated in patients [ 88 ]. Adalimumab (Humira), a human IgG1, has been reported to generate significant immune responses through eliciting anti-idiotypic antibody in a part of patients (5–89%) which varies depending on the disease and the therapy [ 89 , 90 ]. Golimumab (Simponi), a fully human anti-TNFα antibody, combining with methotrexate for treatment of rheumatoid arthritis cause 16% of patients producing anti-drug antibodies [ 91 ]. One reason of these scenarios is that Fv sequence of human antibodies is not identical to human germline: antibody evolution through VJ and VDJ random recombination, as well as affinity maturation naturally occurring in vivo through somatic hypermutation. Until now, there are no in vitro or in-silico assays can precisely analyze the immunogenicity of antibody. In vivo assessments are usually used to evaluate the immunogenicity, of which the result will ameliorate design and engineering of antibody therapeutics to reduce the potential for inducing anti-drug antibodies.
Generation of human antibodies by phage display
Overview of antibody phage libraries.
Phage display is the first and still the most widely used technology for in vitro antibody selection. The strategy was developed based on the excellent work of George P. Smith in 1985 [ 14 ], who used recombinant DNA techniques to fuse foreign peptides with a coat protein (pIII) of bacteriophage M13 in order to display peptides on the bacteriophage surface. He then created “antibody-selectable phage vectors” and described an in vitro method that enabled affinity selection of antigen-specific phage-displayed antibodies from 10 8 -fold excess phage pools [ 92 ]. It was later discovered that scFv, small antibody formats, can be expressed on phage filaments. At the time, there were three different research institutions independently establishing phage-displayed scFv or Fab antibody libraries: the MRC Laboratory of Molecular Biology in the UK [ 13 , 93 , 94 ], the German Cancer Research Center in Germany [ 95 ], and Scripps Research Institute in the USA [ 96 ]. Since then, these phage-displayed antibody libraries have proven to be a reliable discovery platform for the identification of potent, fully human mAbs [ 97 ].
The process of identifying mAbs from a phage-displayed library begins with antibody-library construction (Fig. 4a ). The variable heavy (V H ) and variable light (V L ) polymerase chain reaction (PCR) products, representing the Ig gene-encoding repertoire, are ligated into a phage display vector (phagemid). High quality mRNA from human peripheral blood mononuclear cells (PBMCs) is reverse-transcribed into cDNA. The different V H and V L chain-region gene families are then amplified using specific primers to amplify all transcribed variable regions within the Ig repertoire [ 98 , 99 ]. The format of antibodies in a phage-displayed library can be either scFv or Fab fragments (Fig. 4b ); scFvs are composed of the V H and V L domain connected by a short flexible linker. Antibody Fab fragments displayed on the phage coat protein have comparably higher structural stability and can be readily converted to intact IgG antibodies, usually without impairing binding activity [ 100 , 101 ]. The elegance of phage-displayed libraries is apparent in the linkage between antibody phenotype (specificity and sensitivity) and genotype (genetic information) via the phage particle. Due to the small size and high solubility (10 13 particles/ml) of phage particles, repertoire sizes up to 10 11 independent clones can be efficiently produced and displayed in a single library [ 102 , 103 , 104 ].
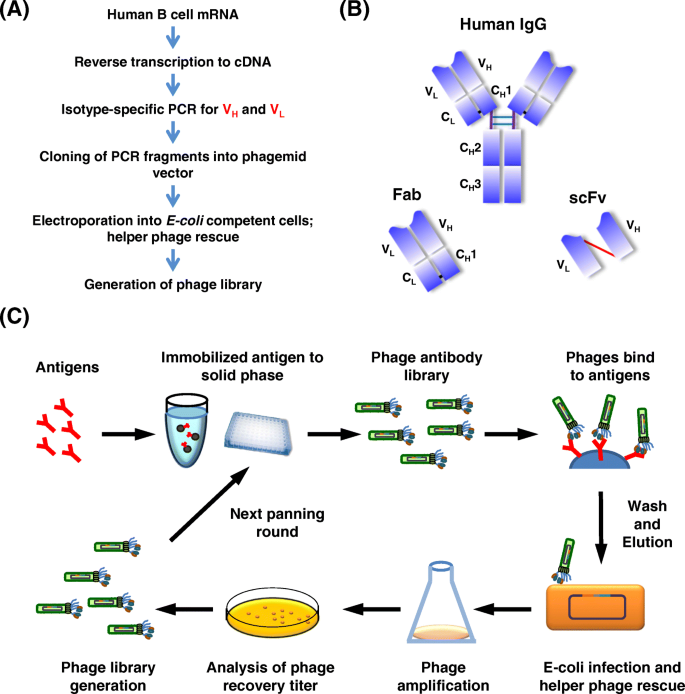
Construction and affinity selection with phage-display antibody library. a Outline of the procedure for constructing a phage-displayed antibody (Fab or scFv) library. b Structure of IgG molecule. Fab consists of the light chain and the first two domains of the heavy chain. scFv is composed of the variable heavy (V H ) and variable light (V L ) domains joined by a short flexible polypeptide linker. c Biopanning with a phage-displayed library. Initial pools of antibodies on the surface of phages are applied to antigens immobilized on a solid surface, e.g., ELISA plates or magnetic beads. Non-specific phages are removed by stringent washing. Antigen-bound phages are eluted and re-infected into E. coli to produce a subset of phages for the next cycle of panning. After several rounds, the antigen-binding clones are sufficiently enriched and individual clones can be selected for further analysis
Gene repertoires for phage display libraries can be obtained from naïve or immunized animals, or the libraries may be synthetically constructed using randomized CDR sequences within fixed frameworks. Phage display naïve antibody libraries are constructed from rearranged V genes of IgM repertoires. Because the gene sequences are derived from B cells of human donors, the naïve libraries are relatively close to the human antibody germ line and have a low risk of immunogenicity. The main advantage of an immunized library over a naïve library is that antibody genes in the immunized library have undergone natural affinity maturation in vivo, allowing the development of high-affinity antibodies against the target. However, this approach requires that immunogenic response can be successfully induced by the antigen of interest, and new libraries must be prepared for each new target. Single large naïve [ 94 , 104 , 105 ] and synthetic [ 102 , 106 , 107 ] libraries have yielded high affinity antibodies (sub-nanomolar range) against a wide spectrum of targets. Therefore, such non-immunized libraries have the distinct advantages of avoiding issues with immunological tolerance in immunized mice, and they do not require new immunized libraries for each new target.
Currently, almost all widely accessible commercial libraries are based on highly diverse non-immunized gene repertoires, which allow selection of antibodies against a virtually unlimited number of targets [ 108 ]. It is worth noting that most antibody drugs that have undergone evaluation in clinical trials originated from a few company-owned libraries. These libraries include: Cambridge Antibody Technology’s (now MedImmune, a subsidiary of AstraZeneca) scFv-fragment library, Dyax Corp’s (now Shire) human Fab-fragment libraries, scFv and Fab libraries from XOMA, and the fully synthetic human combinatorial antibody scFv (HuCAL) and Fab (HuCALGold) libraries developed by MorphoSys [ 97 ].
Affinity selection of human antibodies
Antibody libraries are typically screened by iterative selection cycles to enrich target-binding phages, followed by amplification of the bound phages in E. coli cells. The affinity screening process for antibody libraries is called biopanning (Fig. 4c ). Repeated rounds of selection allow for the enrichment of very rare antigen-binding phage clones, eventually resulting in the selection of the most highly specific binders. This stringent process is a critical feature of phage display that allows mAbs to be isolated in a period as short as a couple of weeks, far more quickly than the traditional hybridoma method [ 99 , 109 ]. For in vitro selection, it is necessary to immobilize target antigens on a solid surface. Polystyrene surfaces with high protein binding capacity, such as 96-well immuno-plates and immuno-tubes, are widely used for antigen immobilization. Additionally, magnetic beads with protein G/A, streptavidin, maleimide or N-hydroxysuccinimide can be used to immobilize antigens and perform biopanning in solution.
The biopanning method is not only restricted to known recombinant proteins. In fact, the phage display technique may also be utilized to select antibodies against whole cells, unveiling previously unknown antigens on the tumor cell surface [ 110 ]. Cancer is an extremely heterogeneous disease, and only a few tumor cells with stem-like properties are able to initiate and sustain tumor development; these cells are often referred as tumor initiating cells or cancer stem cells (CSCs) [ 111 ]. Phage display technology is well suited for applications in CSC research, and several antibodies have already been identified from phage display libraries for their ability to bind known CSC markers, such as CD133 and CD44 [ 112 , 113 ]. Moreover, novel CSC surface markers may be identified by selecting phage-displayed antibodies that bind to a CSC-like population and then identifying the corresponding target antigens [ 114 , 115 ]. The use of tumor biopsy tissue as a biological material allows researchers to probe the tumor microenvironment, which may be highly relevant for clinical use. Phage display technology has been used to probe cancer tissue biopsies in order to generate antibody fragments that specifically recognize tumor subpopulations, such as CSCs and tumor-associated endothelial cells [ 116 , 117 , 118 ] as well as other clinically relevant tumor antigens [ 119 ].
Antibodies or antibody fragments have been referred to as targeted drug delivery “missiles” for their ability to direct homing of drugs to tumors [ 120 ]. For example, immunoliposomes have been demonstrated to provide conventional liposomal drugs with cancer targeting ability, which can increase the therapeutic efficacy of anticancer drugs [ 121 ]. Cellular internalization of the targeting ligand is an essential outcome for successful tumor-targeted liposomal drug delivery [ 99 , 122 ]. For this reason, an efficient phage display-based selection approach was designed to map tumor internalizing epitopes, wherein a phage-displayed library was incubated with living cancer cells at 37 °C [ 123 ]. This method was successfully applied to rapidly identify several scFvs with high rates of internalization in several types of tumors; the target antigens were subsequently identified, and intracellular drug delivery systems were further developed [ 99 , 124 ].
The identification of mAbs with phage display is an entirely in vitro process. Thus, it is not restricted by immunological tolerance, allowing for the identification of antibodies against poorly immunogenic antigens or those that are difficult to obtain using animal immunization methods (e.g., glycans or toxic agents). The in vitro nature of the assay can be especially useful when identifying specific antibodies against novel or gene-mutated pathogens in an outbreak of emergent infectious diseases [ 125 , 126 , 127 ]. The antigens on pathogens usually induce a strong immune response in patients, making it common for infected individuals to naturally produce high-affinity antibodies [ 128 ]. To obtain these antibodies, mRNA from the PBMCs of pathogen-infected people can be quickly collected and used as a gene repertoire for a phage-displayed library [ 129 ]. Such a library can allow for the rapid identification of high-affinity antibodies that may then be used as guides for vaccine design, or to develop therapeutic drugs and diagnostic reagents.
Moreover, the biopanning approach has been modified to isolate and identify a few antibodies with broad neutralizing activity against pandemic influenza virus [ 130 , 131 ]. Chen et al. reported the establishment of a phage-displayed Fab library derived from the PBMCs of convalescent patients infected with a novel influenza A virus H7N9, which broke out in 2013. Using this library, antibodies targeting purified H7N9 virions were isolated [ 132 ]. Two human antibodies were found to exhibit high neutralizing activity against live H7N9 virus due to their interactions with the receptor-binding site of viral hemagglutinin antigens [ 132 , 133 ].
The newly emergent Middle East respiratory syndrome coronavirus (MERS-CoV) induces a severe acute respiratory syndrome-like disease with an approximately 43% mortality rate [ 134 ]. To date, no vaccines or antiviral medications are available for the prevention or clinical treatment of MERS. A large phage-displayed human naive scFv library (Mehta I/II) with 2.7 × 10 10 clones from the Dana-Farber Cancer Institute was used as a resource for the isolation of human antibodies against MERS-CoV [ 135 ]. In another project, a research group in Malaysia improved panning strategies with a naïve human scFv library (library size of 10 9 ) to successfully identify mAbs specific to the MERS-CoV nucleoprotein [ 136 ].
Studies such as those described offer insights into the human antibody response to viral pathogen infection and provide examples of how the outbreak scene may be utilized to develop human antibody-based immunotherapies for the prevention and early treatment of viral pathogens [ 137 ].
The successful development of antibody drugs from phage display
Fully human therapeutic antibodies in current clinical use were discovered from either phage display or transgenic mice approaches [ 138 ]. Phage display has the advantage of allowing researchers to tailor critical characteristics of successful antibody drugs (e.g., affinity, specificity, cross-reactivity and stability). There are nine phage display-derived human antibodies currently approved by the US FDA for the treatment of human disease (Table 1 ), demonstrating the reliability of this technique as a platform for antibody discovery.
Adalimumab (Humira) was developed by BASF Bioresearch Corporation and Cambridge Antibody Technology. It was not only the first phage display-derived antibody granted a marketing approval, but adalimubab was also the first approved (2002) fully human mAb drug [ 139 ]. Adalimumab binds and suppresses TNFα and is approved to treat inflammatory diseases, such as rheumatoid and psoriatic arthritis, Crohn’s disease, and psoriasis. Adalimumab is the world’s best-selling drug [ 140 ] with sales of $19.9 billion in 2018 reported by AbbVie (Table 2 ). Cambridge Antibody Technology also identified human antibodies targeting BLYS (B lymphocyte stimulator) from phage display [ 141 ]. BLYS, a member of the tumor necrosis factor superfamily of cytokines, induces B cell proliferation and differentiation that positively correlate with systemic lupus erythematosus (SLE). This anti-BLYS antibody was named belimumab and marketed as Benlysta by GlaxoSmithKline, becoming the first drug approved (2011) for the treatment of SLE [ 142 ]. Founded in 1989, Cambridge Antibody Technology was acquired by AstraZeneca for $1.32 billion in 2006 [ 143 ].
Tyrosine kinase receptors, including EGFR and vascular endothelial growth factor receptor 2 (VEGFR2), play crucial roles in tumorigenesis, with higher expression and activation in tumors than in normal tissues. These characteristics make the receptors potentially valuable targets for drug development. Necitumumab (Portrazza) is an anti-EGFR human antibody that was identified by screening high EGFR-expressing epidermal carcinoma cells (A431) with a non-immunized phage Fab library of 3.7 × 10 10 clones [ 105 ]. Necitumumab was approved in 2015 and is now a first-line therapy in combination with gemcitabine and cisplatin for the treatment of squamous NSCLC [ 144 ]. VEGFR2 is not only highly expressed in tumor endothelial cells, where it regulates tumor angiogenesis, but it also expressed on the surface of cancer cells. The anti-VEGFR2 human antibody, ramucirumab (Cyramza), was approved for the treatment of gastric cancer, metastatic NSCLC and metastatic colorectal cancer [ 145 , 146 ]. The development of ramucirumab was initiated by using a phage-displayed human naïve Fab library (Dyax) containing 3.7 × 10 10 independent clones for biopanning against the extracellular domain of human VEGFR2 protein [ 105 ]. Three Fab clones, D2C6, D2H2, and D1H4, were selected based on their specific binding to VEGFR2 with nanomolar affinity and their ability to neutralize VEGF-A-activated VEGFR2 signaling. Interestingly, these three Fab clones do not cross-react with murine VEGFR2 and share an identical V H sequence [ 147 ]. After affinity maturation with stringent biopanning rounds, Fab clone 1121 (IMC-1121B) was selected and showed more than the 30-fold improvement of VEGFR2-binding activity. This clone was subsequently engineered into the human intact IgG 1 version (ramucirumab), which has an affinity of 50 pM [ 148 ].
PD-L1, a cell-surface protein, binds to its receptor PD-1 on immune cells, downregulating T cell inflammatory activity to promote self-tolerance by the immune system. Many types of tumors have been found to express PD-L1 on the surface of cancer cells, using the immune-suppressing action to evade immune attacks. Avelumab (Bavencio) is fully human IgG 1 lamda antibody against PD-L1, which was derived from a phage-displayed naïve Fab library (Dyax) [ 149 ]. Avelumab not only blocks PD-L1 binding to PD-1, but it also induces ADCC in cancers [ 150 ]. The later function differs from other immune checkpoint-blocking antibodies. The US FDA approved avelumab in 2017 for the treatment of urothelial carcinoma and Merkel-cell carcinoma, an aggressive type of skin cancer [ 151 ].
Psoriasis is a chronic autoimmune inflammatory disorder that causes skin cell overproduction and is characterized by raised, inflamed, red lesions and plaques that are accompanied by physical pain and itching. Guselkumab (Tremfya) is a fully human antibody developed by Janssen that neutralizes anti-IL-23. The HuCAL antibody library was used to generate guselkumab under a license from MorphoSys [ 152 , 153 ]. In 2017, guselkumab was granted marketing approval by the US FDA for the treatment of plaque psoriasis [ 154 ].
Hereditary angioedema is a rare disease that results in spontaneous, recurrent, and potentially life-threatening attacks of swelling in various parts of the body [ 155 ]. The disease is commonly associated with deficiency or dysfunction of C1-esterase-inhibitor and with excessive bradykinin production caused by overactive plasma kallikrein [ 156 ]. Lanadelumab (Takhzyro) is a fully human mAb derived from the Dyax phage library; it directly binds the active site of plasma kallikrein to inhibit bradykinin production. Lanadelumab was approved in 2018 in the USA and Canada for prophylaxis against attacks of hereditary angioedema in patients aged ≥ 12 years [ 157 ].
The success of a drug development effort is highly dependent on obtaining patent protection for products and technologies while avoiding infringement on patents issued to others. Therefore, intellectual property rights for phage-display antibody discovery platforms comprise a changing landscape that greatly affects drug development. Currently (2019), almost all of the key patents regarding phage display technologies have expired, including the Breitling/Dübel (EP0440147) and McCafferty/Winter (EP0774511, EP0589877) patents that expired in 2011 in Europe [ 149 ]. The US patents covering Dyax and Cambridge Antibody Technology phage antibody libraries have also reached the end of their 20-year protection period (Table 3 ). The expiration of these patents will allow more companies to create phage display human antibody libraries, advancing the march of therapeutic antibodies into the clinic. The lifting of intellectual property constraints will also spur academic institutions to translate developed phage-displayed antibodies into the clinic.
Many researchers have begun to take advantage of these free technologies. For example, Wayne Maraso and colleagues at the Dana-Farber Cancer Institute in Harvard University have constructed two phage display libraries containing 12 billion (Mehta I) and 15 billion (Mehta II) human naïve scFv antibody phages. These libraries have been used to identify numerous human scFv antibodies against a variety of targets [ 158 , 159 ]. James Marks’ group has also established a phage-displayed human scFv library containing 6.7 billion members at the University of California, San Francisco [ 98 ]. This library has yielded a panel of specific antibodies for membrane proteins and living tissues with sub-nanomolar affinity [ 98 , 160 , 161 ]. We have also established a phage-displayed human naive scFv library at the Institute of Cellular and Organismic Biology (ICOB) in Academia Sinica in Taiwan. The antibody gene repertoires of the ICOB phage antibody library were isolated from the PBMCs of 50 healthy human donors, producing a library size of 60 billion individual scFv clones. This collection has been successfully used to select antibodies that bind a wide spectrum of target antigens, including pure recombinant proteins, glycans, cancer cells and virus particles [ 99 , 103 , 104 ].
Human antibody-producing mice
Transgenic animals provide a reliable platform for antibody drug development. Compared with other technologies for human antibody production, transgenic animals have several advantages, i.e., no need for humanization, more diversity, in vivo affinity maturation and clonal selection for antibody optimization. However, the large size of human Ig loci was a challenge during the development of transgenic mouse antibody technology. Additionally, the production of repertoires in transgenic mice that are similar or comparable those in humans requires diverse rearrangements combined with high expression of human V, D, and J segments [ 162 ]. To overcome these major challenges, different strategies have been successfully used to generate animals expressing human antibody repertoires (Table 4 ) [ 35 , 36 , 165 ].
Fully human antibody mice
The idea of producing human antibodies in transgenic mice was first suggested in 1985 when Alt et al. [ 166 ] proposed introducing human antibody genes into the mouse germline. This idea was unprecedented and provided a new direction for the development of human antibody production. In 1989, Brüggemann et al. [ 167 ] cloned the first human heavy chain construct, containing two human V H genes, for diversity segments (D) linked to the human heavy chain joining cluster (J H ), and the μ constant region. The 25 kb construct was micro-injected as a mililocus plasmid into fertilized murine eggs, allowing its random insertion into the murine genome. About 4% of B lymphocytes expressed human μ chain at detectable levels in these transgenic mice. In addition, hybridomas of human IgM antibody could also be established using this transgenic strain. In 1992, Taylor et al. cloned the human κ light chain [ 168 ] construct, containing one human kappa light chain variable (Vκ) gene, the human kappa light chain joining cluster (Jκ) and kappa constant region (Cκ). While the mice expressed the human heavy chain (V H -D-J H -Cμ-Cγ 1 ) and the human kappa light chain, the amount of human antibody was less than 10% of total antibodies, so the expression of human antibodies was not compatible with expression of mouse endogenous Ig [ 168 ].
At a similar time, various murine Ig knockout mouse strains were generated. In 1993, Chen et al. knocked out murine J H and Jκ genes with gene targeted deletion, inactivating mouse Ig [ 169 , 170 ]. The human IgH and IgL transgenic mice were then crossed with murine IgH and IgL knockout mice in an attempt to create lines that could generate more diverse human antibodies. In 1994, the first human Ig -transgenic mice strain, HuMabMouse [ 35 ], was generated by Longberg et al. In this line, human IgH and IgΚ are expressed in murine IgH and IgΚ deficient mice. The entire human IgH genome is about 1.29 Mb and IgΚ is about 1.39 Mb, but the human Ig genome introduced into mice was less than 80 kb [ 35 ]. Since antibody diversity comes from germline V(D) J genes, it is reasonable that the introduction of more human variable genes will lead to more diversity of generated antibodies.
Likewise, Davies et al. [ 171 ] and Choi et al. [ 172 ] used yeast artificial chromosome (YAC) vectors in 1993 to respectively construct human IgΚ (~ 300 kb) and IgH (~ 85 kb) genes via yeast homologous recombination. In 1994, Green et al. [ 37 ] constructed human IgΚ (~ 170 kb) and IgH (~ 220 kb) genome YACs and successfully introduced them into mouse embryonic stem cells by yeast spheroplast-ES cell fusion. Furthermore, in 1997, Mendez et al. [ 36 ] introduced a larger human IgΚ (~ 700 Kb) or IgH (~ 1 Mb) YAC into mouse ES cells, crossing the human Ig mice with murine IgH and IgL knockout mice to generate XenoMouse [ 131 ]. As expected, the XenoMouse only express human antibodies rather than mouse antibodies [ 173 ]. While the development of this line has eliminated the interference from mouse endogenous Ig and expanded the human Ig genes, the efficiency of human antibody generation, Ig class-switching and somatic hypermutation still remain low, due to a lack of mouse constant region gene expression [ 158 ].
Chimeric human antibody mice
In order to overcome the drawbacks of a fully human heavy chain antibody, it is necessary to retain the original murine constant region. If a chimeric antibody with human Fab and murine Fc can be generated in mouse, the murine Fc would modulate the signaling for somatic hypermutation during antibody affinity maturation [ 158 , 174 , 175 ] and effector function of antibodies [ 174 , 176 ]. Along these lines, Osborn et al. [ 163 ] linked human V H , D, and J H genes to the rat constant region locus in 2013. The large segments of human IgH and IgL were subcloned and linked by bacterial artificial chromosome (BAC) and YAC techniques, followed by micro-injection of the minilocus plasmid into fertilized rat oocytes. Meanwhile, endogenous rat Ig loci were silenced with a zinc finger nuclease. The resulting rat strain (OmniRat) chimeric human exhibits antibody production, antigen affinity and somatic mutations similar to wild-type rats. In 2014, Lee et al. [ 164 ] utilized BACs with Cre/loxP recombination in mouse ES cells to generate a mouse strain with human V H -D-J H and Vκ-Jκ inserted directly upstream of murine Cμ and Cκ regions (KyMouse). After antigen immunization, the KyMouse can process somatic hypermutation and produce high-affinity chimeric human antibodies. In another effort, Murphy et al. [ 165 ] used BACs to assemble large human Ig genes and serially micro-injected the constructs into mouse ES cells. The human IgH and IgL genes targeted and replaced the murine IgH and IgL, upstream of the constant region (VelocImmune mouse).
Development of successful antibody drugs from human antibody mice
Generation of human antibodies by transgenic animals has been accomplished by seven companies: Abgenix, XenoMouse (purchased by Amgen in 2005); Medarex, HuMAbMouse (purchased by Bristol Myers Squibb in 2009); Ligand, OmniRat; Kymab, KyMouse; Regeneron VelocImmune mouse; and the more recent Harbour Antibodies, H2L2 Mouse and Trianni Inc., Trianni Mouse [ 162 , 165 ] (Table 4 ). The first antibody drug derived from transgenic mice was approved by the US FDA in 2006 [ 177 ], and as of 2019, 19 transgenic animal-derived antibody drugs [ 17 , 18 , 19 , 20 , 21 , 22 , 23 , 24 , 25 , 26 , 27 , 28 , 29 , 30 , 31 , 32 , 33 , 34 , 177 ] generated by Xenomouse, HuMabMouse, and VelocImmune mouse are on the market (Table 5 ). Eight of the drugs are used for cancer treatment, while the others are for autoimmune or inflammatory diseases.
There have been seven US FDA-approved antibody drugs generated from the XenoMouse (Table 5 ). In 2006, the first one, panitumumab (Vectibix, Amgen, human IgG2/kappa), was approved to treat the EGFR-expressing metastatic colorectal cancer with wild-type KRAS [ 188 ]. This mAb blocks the interaction of EGFR and its ligands, resulting in the inhibition of EGFR signaling and induction of cancer cell apoptosis. Two antibody drugs from the XenoMouse are used for autoimmune dermatologic diseases. One, secukinumab (Cosentyx, Novartis, human IgG1), binds to proinflammatory cytokine IL-17α to reduce inflammation in psoriasis [ 189 ]. The other is brodalumab (Siliq, Valeant Pharmaceuticals, human IgG2), which binds to the IL-17 receptor to inhibit the action of IL-17 family cytokines. The two mAb drugs were approved by the US FDA for psoriasis treatment in 2015 and 2017, respectively.
From the HuMabMouse, there have also been eight antibody drugs approved by the US FDA (Table 5 ). Two drugs, ipilimumab (Yervoy, Bristol-Myers Squibb, human IgG1) and nivolumab (Opdivo, Bristol-Myers Squibb, human IgG4/kappa), are used for melanoma treatment; the drugs were approved in 2011 and 2014, respectively. Ipilimumab binds to CTLA-4, an immune checkpoint inhibitor, blocking its interaction with B7 on APCs and causing cytotoxic T lymphocytes to kill cancer cells [ 190 ]. Nivolumab recognizes to PD-1, reducing inhibitory signaling to rehabilitate the immune response of tumor-specific T cells in patients [ 191 ]. Notably, nivolumab was also approved for non-small cell lung cancer treatment in 2018. Among the mAb drugs derived from the HuMabMouse, some are used for autoimmune diseases. For example, ustekinumab (Stelara, Johnson & Johnson, human IgG1/kappa) binds to cytokines, especially the p40 subunits of IL-12 and IL-23, blocking proinflammatory signaling to ease inflammation. This drug was approved for severe plaque psoriasis [ 17 ] in 2009 and for Crohn’s disease [ 192 ] in 2016.
The VelocImmune mouse is a second generation transgenic chimeric mouse and has yielded four approved drugs (Table 5 ). Dupilumab (Dupixent, Sanofi and Regeneron, human IgG4) binds to IL-4 receptor and inhibits the IL-4 and IL-13 pathway, as an eczema treatment. Sarilumab (Kevzara, Sanofi and Regeneron, human IgG1) inhibits IL-6 signaling by binding to the IL-6 receptor (IL-6R), which otherwise would upregulate the release of rheumatoid arthritis-related factors from hepatocytes. The two drugs were both approved in 2017. Notably, despite having access to the XenoMouse and owning Cambridge Antibody Technology (the phage display company behind Humira), AstraZeneca paid over $120 million for a few breeding pairs of VelocImmune mice [ 193 ].
To improve the diversity of products and generate better antibody drugs, major development efforts have yielded models, such as the fully human antibody mouse and second-generation chimeric human antibody mice, over the 30 years since the first transgenic mouse was generated in 1989 [ 167 ]. The continued refinement and advancement of transgenic animals provides ever more possibilities for antibody drug development by global pharmaceutical factories.
- Single B cell antibody technology
In the human immune system, antibody responses are robust, highly specific, neutralizing and self-tolerant. Producing therapeutic human antibodies using the traditional hybridoma technique or transgenic mice requires long-term immunization procedures and screening, while the clinical use of murine antibodies may trigger severe immunogenic responses (such as HAMA) [ 194 ]. To avoid these obstacles, a technique for immortalizing human B cells with Epstein-Barr virus was developed [ 195 , 196 , 197 ]. This method is useful in certain situations, but it suffers from drawbacks, such as inefficiency in some patients and difficulties maintaining the stability of some transformed clones. While mice carrying human Ig genes have been created [ 35 , 36 , 163 , 164 , 165 ], the immune reactivity of these mice often cannot be triggered as robustly as natural human antibody responses. Thus, in emergent cases such as infectious diseases, single B cell antibody technologies have the major advantage of requiring only a few cells, allowing the highly efficient and rapid isolation of potential mAbs. Moreover, single B cell cloning preserves the biologically mediated heavy chain and light chain pairing, instead of the random pairing that is characteristic of mAbs from phage display antibody libraries. These randomly paired mAbs occasionally lose binding affinity or develop self-reactivity when transferred from scFv to intact IgG formats.
Identification and isolation of single B cells
Single B cells can be isolated from either PBMCs or lymphoid tissues using micromanipulation [ 198 , 199 ], laser capture microdissection [ 200 ], and fluorescence-activated cell sorting [ 199 , 201 , 202 ]. Generally, mononuclear cells are purified from PBMCs or bone marrow by Ficoll-Paque density gradient centrifugation. Based on B cell expression of specific cell surface markers in different stages, isolation of single B cells by fluorescence-activated cell sorting is widely utilized, especially in the identification of rare and discrete B cell subpopulations. Antigen-coated magnetic beads [ 203 ] and fluorescence-conjugated antigens [ 204 , 205 , 206 ] are also often used to select antigen-specific B cells in a process known as antigen baiting. Neutralizing human mAbs against Puumala virus were generated from B cells isolated using antigen-coated magnetic beads [ 207 ]. Recently, antigen-conjugated fluorescent beads have been used to identify antigen-specific B cells [ 208 ]. Fluorescent virus-like particles of rotavirus served as antigen bait for single RV-specific B cells, which had been extracted from healthy rotavirus-infected infants or adult donors [ 209 ]. HIV envelope protein antigens have also been used to isolate antibodies that broadly neutralize HIV-1 [ 210 , 211 ]. Moreover, isolation of dengue virus-specific memory B cells was reported [ 212 , 213 ]. Thus, antigen baiting may be applied as a preliminary selection tool to be used on a polyclonal mixture.
Cloning of single B cells and screening of antibodies
After single B cell sorting, direct cloning of each Ig heavy chain and corresponding light chain should be performed [ 201 , 202 ]. This step involves the use of nested or semi-nested reverse transcription-polymerase chain reaction (RT-PCR) for amplification of the variable heavy and light chain of each identified B cell. Usually, forward primers are directed toward IgH and IgL variable leader sequences and reverse primers are complementary to the Ig constant region [ 201 , 214 ]. By optimizing different primer-set mixtures, the recoveries of the V H and V L may be improved [ 201 , 215 ]. The genes are then cloned and expressed in mammalian cell lines for the immediate generation of recombinant mAbs. Following the detection of mAb reactivity, the characteristics of each generated mAb are determined. Furthermore, for high-throughput screening and evaluation of secreted mAbs with ideal reactivity, a cell-based microarray chip system [ 216 ] and microengraving techniques [ 217 , 218 , 219 , 220 ] have been described. The cell-based microarray chip system, immunospot array assay on a chip, enables the trapping of secreted antibodies by a chip that is coated with antibody against Ig, therefore, it is used to identify and recover specific antibody-secreting cells [ 216 ]. The microengraving method depends on the use of a soft lithographic technique to generate microarrays comprising the secreted antibodies of single cells [ 217 ]. These two approaches offer the advantages of early and rapid identification of clones with high affinity and specificity to the antigen of interest.
Generation of human antibodies by single B cell
In the face of threats from novel emergent pathogens, the rapid development of immunotherapies or insights into the diversity of antibody repertoire are beneficial, and single B cell sorting provides a highly efficient technology to achieve these goals. In the past, human mAbs have been generated by the single B cell method for bacterial, parasitic, virus infected or autoimmune diseases.
Among bacteria, Bacillus anthracis is one of the most concerning species. B. anthracis is a fatal pathogen that causes severe anthrax disease in humans and has been used as a biological weapon. Although antibiotics are available for anthrax treatment and as post-exposure prophylaxis, anti-anthrax protective antibodies from single human B cells will still be a crucial addition to the treatment toolkit [ 221 , 222 ]. In an example targeting yeast infections, anti- Candida mAbs antibodies derived by the single human B cell method can enhance phagocytosis to protect against disseminated candidiasis [ 223 ].
The single B cell method has also successfully yielded anti-viral mAbs. Rapid isolation of dengue-neutralizing antibodies from human antigen-specific memory B-cell cultures [ 224 ] and characterization of antigen-specific B cells in the peripheral blood of DENV-immune individuals [ 213 ] were both reported. In another example, Iizuka and colleagues described the identification of cytomegalovirus pp65 antigen-specific human mAbs using single B cell-based antibody gene cloning [ 225 ]. For rotavirus, the single B cell method was performed to analyze the rotavirus antibody gene repertoire of VP6-specific B cells in naive and memory B cell subsets [ 226 ] and generate rotavirus-specific human mAbs by sorting single B cells from small-intestinal mucosa [ 227 ]. Human mAbs against zika virus NS1 have also been generated by the single B cell method [ 228 ]. Besides mAbs for bacterial and virus infection, the single human B cell method has also yielded a complement factor H (CFH) therapeutic antibody for cancer. The recombinant anti-CFH antibody can induce complement-mediated cytotoxicity (CDC) through complement activation and release of anaphylatoxins [ 229 ].
Development of single B cell-derived antibodies in clinical trials
Many virus-targeting mAbs are also currently in clinical trials. For example, Ebolavirus is a highly lethal pathogen that causes 25–90% mortality in humans. Therapeutic mAbs for ebolavirus infection have been derived from B cells of vaccinated human donors or survivors [ 230 , 231 , 232 ]. Impressively, human mAb114, which is derived from sorted memory B cells targeted to the Zaire ebolavirus glycoprotein, protects macaques when administered as late as 5 days after challenge [ 231 ]. Clinical trials for this drug are at Phase I (NCT03478891), Phase II and III (NCT03719586).
Acquired immunodeficiency syndrome is caused by HIV, and an estimated 36.9 million people worldwide are infected. HIV-1 envelope protein is an attractive therapeutic target for antibody and vaccine design. Five human mAbs against anti-HIV envelope protein have been generated by the single B cell approach and are under evaluation in clinical trials (3BNC117, Phase I/II; 10–1074, Phase I; VRC01, Phase I/II; PGT121, Phase I/II and N6, Phase I) [ 233 ]. A Phase I clinical trial (NCT02579083) is also being conducted on the prevention of sexual transmission of HIV-1 and herpes simplex virus by MB 66 combined with an anti-herpes simplex virus antibody (AC8) and an anti-HIV antibody (VRC01).
Different influenza viruses cause epidemics ever year, and influenza vaccines are the most useful measure to prevent seasonal influenza. Single B cell isolation for the generation of potent and broadly neutralizing anti-influenza antibodies has become a popular undertaking [ 234 , 235 ]. MHAA4549A, a human mAb targeting the hemagglutinin stalk of influenza A virus was cloned from a single human plasmablast cell from an influenza virus vaccinated donor [ 236 ]. A Phase II clinical trial of MHAA4549A as a monotherapy for acute uncomplicated seasonal influenza A in otherwise healthy adults was recently completed (NCT02623322). CT P27, which contains two human mAbs (CT P22 and CT P23), was created by Celltrion and is in Phase II (NCT03511066). RG 6024, also named MHAB5553A, was generated by Genentech with a modified version of the single B cell isolation method [ 237 ]; it is currently under examination in Phase I (NCT02528903) trial. The Phase II trial for another antibody, TCN 032, was stopped in 2012 (NCT01719874).
Profiling of respiratory syncytial virus (RSV) antibody repertoires from the memory B cells of naturally infected adults [ 238 ] or generation of neutralizing antibodies from RSV-infected infants [ 239 ] has been undertaken as well. MEDI8897, an anti-RSV antibody developed by MedImmune, is currently being evaluated for safety and efficacy in Phase II clinical trial (NCT02878330).
Over the past decade, generation of mAbs by single B cell technology has become increasingly attractive. However, there are still no US FDA-approved therapeutic mAbs developed by this method that is used for clinical treatment of any disease. Although single B cell technology possesses several irreplaceable advantages, there are still challenges to be overcome. For example, the antigen labeling technique, the configuration of sorting antigens (e.g., monomer or dimer) and the design of primer sets are all important considerations for successful generation of mAbs. In the future, recovery of mAbs from single B cell platforms may be a powerful tool in combination with next generation sequencing for development of novel diagnostics, pharmacokinetic applications, and clinical therapeutics.
Affinity maturation of antibodies
Antibodies identified from humanized, phage or transgenic methods are often further engineered, including the replacement of residues with binding liabilities in the binding region. In addition, point mutations in the antibody structure sometimes result in products with weaker antigen interactions than the original antibody (low affinity), but some mutations will result in stronger interactions (high affinity). The process of enhancing affinity for antigens is called affinity maturation. After V(D) J recombination, affinity maturation occurs in mature B cells with the help from helper T cells.
Affinity maturation is an important characteristic of the humoral immune response, which can result in antibodies with low picomolar affinity [ 240 , 241 ]. High affinity is a crucial attribute of antibodies for the neutralization of cytokines or growth factor-induced signaling. Generally, for a mAb to be considered for therapeutic drug development, it should have an affinity of 1 nM or less for the target antigen [ 242 ]. Moreover, humanization of mouse mAbs frequently reduces affinity [ 243 ]. Thus, the use of affinity maturation is often a necessary step in antibody drug development [ 244 ].
Approaches for affinity maturation
Phage display and yeast display have been widely used for affinity maturation of antibodies, due to their amenability to easily screen for high affinity variants and to high throughput applications [ 81 ]. Methods for increasing antibody affinity can be broadly divided into two broad categories. The first is to generate a large randomly mutated library of CDR or entire variable domain sequences, followed by a selection of higher affinity variants from this large number of mutants. Another approach is to prepare small libraries by focused mutagenesis or hotspot mutagenesis that mimics in vivo affinity maturation. In this focused method, a high affinity variant is selected to be randomized at individual positions in each of the six CDRs or at a discrete point in the variable domain called the hotspot. The usual practice is to combine different mutations, resulting in small increases in affinity. The combination of these different mutations may have an additive or synergistic effect that can result in substantial increases in the affinity of the antibody to the antigen [ 245 ]. Phage display technology can be employed to identify high affinity antibodies in an antibody gene mutation library under stringent biopanning conditions, including decreased antigen amount, extended incubation and intensive washing steps or by competition with soluble antigen [ 104 , 246 ]. Using phage display with V H and V L CDR3 mutation libraries, the affinity of anti-HER2 antibody was improved more than 1200-fold [ 97 ].
Diversification of antibody genes is the initial step of affinity maturation in vitro, and this step may be achieved using various strategies, such as random mutations, targeted mutations or chain shuffling [ 246 ]. Mutations can be randomly introduced into the variable regions of antibody genes by error-prone PCR in mutator E. coli strains [ 247 , 248 ]. Chain shuffling approaches are those in which one of two chains, V H and V L , is fixed and recombined with a repertoire of partner chains to produce a next-generation library [ 249 ]. Moreover, mutations can be introduced to particular regions of the antibody gene. This type of targeting mutation approach was employed to diversify CDR residues and was shown to be effective in ameliorating the affinity of antibodies [ 250 ]. Therefore, this method is more relevant to in vivo somatic mutations during B cell evolution because mutations accumulate more efficiently in the CDR than framework residues.
Future perspectives
The field of therapeutic antibodies has undergone rapid growth in recent years, becoming a dominant force in the therapeutics market. However, there is still significant growth potential for the therapeutic antibody field. Traditionally, antibodies have been used for the treatment of cancer, autoimmune diseases, and infectious diseases. If the molecular mechanisms of a specific disease can be clearly elucidated and the specific proteins or molecules involved in pathogenesis can be identified, antibodies may provide an effective therapeutic option. For example, anti-CGRP receptor antibodies (erenumab, galcanezumab, or fremanezumab) have been developed for the prevention of migraine. Anti-proprotein convertase subtilisin/kexin type 9 (PCSK9) antibodies (evolocumab or alirocumab) are used for the treatment of hypercholesterolemia. Anti-fibroblast growth factor 23 (FGF23) antibody (burosumab) is used to treat X-linked hypophosphatemia. Anti-IL6R antibody (sarilumab and tocilizumab) can be used for the treatment of rheumatoid arthritis. Anti-Factor IXa/Xa antibody (emicizumab) is a valuable treatment for hemophilia A. Anti-von Willebrand factor antibody (caplacizumab) is approved for the treatment of thrombotic thrombocytopenic purpura, and other antibodies will be approved for new indications in the near future.
Therapeutic antibodies can roughly be separated into two broad categories (Fig. 5 ). In the first category, the naked antibody is directly used for disease therapy. Cancer treatments from this category may act through several different mechanisms, including mediated pathways (e.g., ADCC/CDC), direct targeting of cancer cells to induce apoptosis, targeting the tumor microenvironment, or targeting immune checkpoints. In mediated pathways, the antibody kills cancer cells by recruiting natural killer cells or other immune cells. Recently, new technological developments have been made to enhance the therapeutic effects of ADCC or CDC, such as antibody Fc point mutations [ 251 , 252 , 253 ] or modification of glycosylation [ 254 , 255 , 256 , 257 , 258 ] to improve cancer cell killing capabilities. The direct induction apoptosis in cancer cells has traditionally been the preferred mechanism for therapeutic antibodies. With regard to targeting the tumor microenvironment, antibodies can inhibit tumorigenesis by targeting factors involved in cancer cell growth. For example, Avastin targets vascular endothelial growth factor (VEGF) to inhibit blood vessel growth around the tumor, shutting down the supply of nutrients required for the cancer cell growth. Immune checkpoints have proven to be valuable targets for cancer treatment. In the future, studies evaluating synergistic effects of antibodies and chemotherapeutic drugs, radiotherapy or other biologic agents will greatly benefit the further development of antibody therapeutics. Furthermore, the identification of novel biomarkers may improve the efficacy and specificity of antibody-based therapy for human diseases.
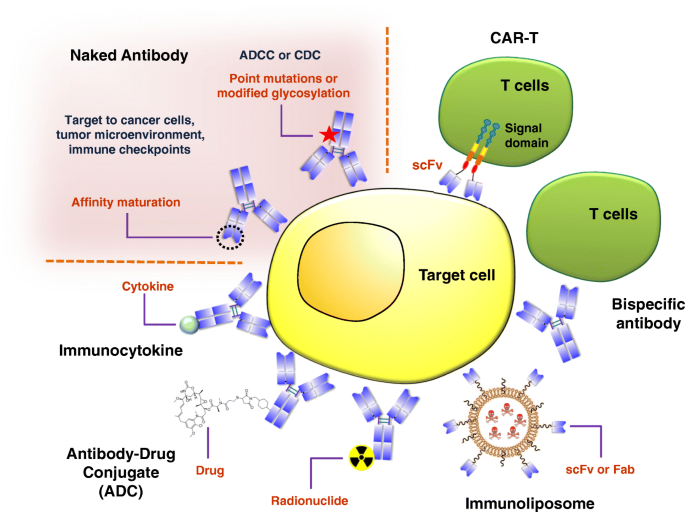
Schematic overview showing the development of antibody-based therapeutics for the treatment of cancer. Therapeutic antibodies can be roughly separated into two broad categories. The first category involves the direct use of the naked antibody for disease therapy. Antibodies in this category are used for cancer treatment and elicit cell death by different mechanisms, including ADCC/CDC, direct targeting of cancer cells to induce apoptosis, targeting the tumor microenvironment, or targeting immune checkpoints. For antibodies in the second category, additional engineering is performed to enhance their therapeutic efficacy. Some general approaches for the use of these antibodies include immunocytokine, antibody-drug conjugate (ADC), antibody-radionuclide conjugate (ARC), bispecific antibody, immunoliposome, and CAR-T
In the second category of antibody drugs, additional modifications are made to the antibody in order to enhance its therapeutic value. Some general approaches include immunocytokines, antibody-drug conjugate, antibody-radionuclide conjugates, bispecific antibodies, immunoliposomes, and chimeric antigen receptor T cell (CAR-T) therapy. To create an immunocytokine, a selected cytokine is fused to an antibody to enhance delivery specificity [ 259 ]. Antibody drug conjugates consist of an antibody that targets a cancer-specific marker conjugated to the small molecule drug; the antibody enhances delivery to the tumor site, increasing the efficacy of the small molecule while reducing side effects by reducing non-specific toxicity to non-target tissues [ 260 ]. The antibody may also be conjugated to a radionuclide, in order to direct radiotherapy more specifically to the tumor site [ 261 ]. For bispecific antibodies, antibodies targeting two receptors are engineered to further enhance therapeutic effects [ 262 ]. Antibody-engaged effector cell functions may enhance the therapeutic efficacy of bispecific antibodies. With regard to immunoliposomes, the binding site of the antibody (scFv or Fab) is cleaved from the constant region and subsequently conjugated to different nano-drug delivery systems, such as liposomal drugs, to provide more specific targeting [ 263 , 264 ]. Lastly, CAR-T involves inserting the gene for a chimeric T cell receptor-antibody targeting a specific cancer marker into T cells, such that the engineered cells target and kill cancer cells [ 265 , 266 ]. In recent years, this approach has garnered major attention from the scientific and medical community due to its significant clinical benefits to cancer patients. In many cases, patients have experienced complete remission or even been completely cured of cancer [ 267 , 268 , 269 , 270 , 271 ].
Although new methods have been well-established for generating fully human antibodies, such as human antibody transgenic mice and human single B cell antibody techniques, phage display still has advantages as an antibody drug discovery platform, based on its efficient and economical in vitro selection methodology. Recently, some advanced techniques have been applied in antibody discovery, including high-throughput robotic screening [ 272 ], next generation sequencing [ 273 ] and single cell sequencing [ 274 , 275 ]. These techniques are expected to greatly accelerate the identification of specific phage binders, facilitating mAb development for use in research, clinical diagnostics, and pharmaceuticals for the treatment of human disease.
By reviewing currently approved mAbs, one may easily see how sophisticated formats were developed in response to challenges posed by therapeutic indications. These mAb engineering solutions are highlighted by antibody-drug conjugates, glycoengineered mAbs, immunomodulators, bispecific mAbs, and CAR-T cells.
Conclusions
Here, we summarize five technical platforms that are related to the production of therapeutic antibodies, including chimeric antibodies, humanization, phage display, transgenic mice, and single B cell antibody technology (Fig. 3d ). Phage display, transgenic mice, and single B cell antibody technology have proven to be reliable methods for the generation of human antibodies. As enormous storehouses of antibody encoding genes (> 10 10 ) with unknown properties, high quality (antibody diversity) phage antibody libraries are critical to the successful identification of therapeutic mAbs. In addition, an optimal selection from phage display libraries is dependent on target antigen quality, antigen immobilization, and tight control of binding and wash conditions. Furthermore, careful pre-screening design of conditions can tailor the characteristics of antibodies discovered from biopanning, including conformation specificity, epitope specificity, internalization, neutralization, and interspecies cross-reactivity. Currently, there are nine fully human antibodies that were discovered from phage libraries approved for therapy, and dozens more phage-derived antibody therapeutics are in clinical trials, waiting to enter the market [ 149 ] (Table 5 ).
In order to improve the quality of antibody drugs, researchers have developed several transgenic animals, including fully human mice and second-generation human chimeric mouse. The continued refinement and advancement of transgenic animals provide more options for antibody drug development in global pharmaceutical factories. All the transgenic-derived mAbs approved for therapeutic use have come from from three companies: Abgenix (XenoMouse) [ 36 ], Medarex (HuMAbMouse) [ 35 ], and Regeneron (VelocImmune) [ 165 ]. Depending on the immunization protocol, high affinity human antibodies can be obtained through selection of the clones generated in the animals. This selection is mainly accomplished by hybridoma technology. Currently, there are 19 approved human mAbs that were discovered from these three transgenic animals (Table 5 ).
Over the past decade, generation of mAbs from isolated single B cells has become an increasingly attractive approach. To date, no US FDA-approved therapeutic mAbs have been derived from this method; however, it possesses several major advantages, and ongoing challenges are currently being solved. The success of the method relies heavily on the antigen labeling technique, the configuration of sorting antigens (e.g., monomer or dimer) and the set of primers used for amplification. In the future, the recovery of mAbs from single B cells is expected to become a powerful tool in combination with next generation sequencing for diagnostics, pharmacokinetic application, and clinical therapeutics.
As a result of highly active development of antibody drugs in recent decades, mAbs have emerged among the major class of therapeutic agents for the treatment of many human diseases, especially cancers, immunological, infectious, neural and metabolic diseases. Sales growth and regulatory approval of mAb products were slow until the late 1990s when the first chimeric mAbs were approved (annual sales of $0.3 billion in 1997). With the subsequent approval of humanized and then fully human mAbs, the rate of product approvals and sales of mAb products has increased rapidly, with global sales revenue for all mAb products at $115.2 billion in 2018 (Fig. 1 ) [ 276 ]. The continued growth of mAb products in the coming years is expected to be a major driver of overall biopharmaceutical product sales.
Availability of data and materials
Not applicable.

Abbreviations
Antibody-dependent cellular cytotoxicity
Bacterial artificial chromosome
B lymphocyte stimulator
Calcitonin receptor-like receptor
Chimeric antigen receptor T cell
Complement-mediated cytotoxicity
Complementary-determining region
Calcitonin gene-related peptide
Chronic lymphocytic leukemia
Coronavirus
Cancer stem cell
Cytotoxic T-lymphocyte–associated antigen 4
Κappa constant region
Diversity segment
Epidermal growth factor receptor
Embryonic stem cell
Antigen-binding fragment
Fragment crystallizable region
Human anti-mouse antibody
Human epidermal growth gactor receptor 2
Human immunodeficiency virus
Institute of Cellular and Organismic Biology
Immunoglobulin
Heavy chain joining cluster
Kappa chain joining cluster
Monoclonal antibody
Middle East respiratory syndrome
Multiple myeloma
Microsatellite instability high
Non-small cell lung cancer
Peripheral blood mononuclear cell
Polymerase chain reaction
Programmed cell death protein 1
Programmed death-ligand
Receptor activity-modifying protein 1
Respiratory syncytial virus
Reverse transcription-polymerase chain reaction
Single chain fragment variable
Systemic lupus erythematosus
Tumor necrosis factor α
United States Food and Drug Administration
Vascular endothelial growth factor receptor 2
Variable heavy chain
Variable light chain
Kappa variable light chain
Yeast artificial chromosome
Kohler G, Milstein C. Continuous cultures of fused cells secreting antibody of predefined specificity. Nature. 1975;256:495–7.
Article CAS PubMed Google Scholar
Kaplon H, Reichert JM. Antibodies to watch in 2019. MAbs. 2019;11:219–38.
The Antibody Society. In: Approved antibodies. Jun 27, 2019 https://www.antibodysociety.org/ Accessed 15 Jul 2019.
Lefranc MP. IMGT, the International ImMunoGeneTics Information System. Cold Spring Harb Protoc. 2011;2011:595–603.
PubMed Google Scholar
Ecker DM, Jones SD, Levine HL. The therapeutic monoclonal antibody market. MAbs. 2015;7:9–14.
Morrison SL, Johnson MJ, Herzenberg LA, Oi VT. Chimeric human antibody molecules: mouse antigen-binding domains with human constant region domains. Proc Natl Acad Sci U S A. 1984;81:6851–5.
Article CAS PubMed PubMed Central Google Scholar
Foster RH, Wiseman LR. Abciximab. An updated review of its use in ischaemic heart disease. Drugs. 1998;56:629–65.
Maloney DG, Grillo-Lopez AJ, White CA, Bodkin D, Schilder RJ, Neidhart JA, et al. IDEC-C2B8 (Rituximab) anti-CD20 monoclonal antibody therapy in patients with relapsed low-grade non-Hodgkin's lymphoma. Blood. 1997;90:2188–95.
Maloney DG, Grillo-Lopez AJ, Bodkin DJ, White CA, Liles TM, Royston I, et al. IDEC-C2B8: results of a phase I multiple-dose trial in patients with relapsed non-Hodgkin's lymphoma. J Clin Oncol. 1997;15:3266–74.
Jones PT, Dear PH, Foote J, Neuberger MS, Winter G. Replacing the complementarity-determining regions in a human antibody with those from a mouse. Nature. 1986;321:522–5.
Tsurushita N, Hinton PR, Kumar S. Design of humanized antibodies: from anti-Tac to Zenapax. Methods. 2005;36:69–83.
Watier H, Reichert J. Evolution of antibody therapeutics. In: Vaughan T, Osbourn J, Jallal B, editors. Protein terapeutics. Weinheim: Wiley-VCH Verlag GmbH & Co, KGaA; 2017. p. 25–49.
Chapter Google Scholar
McCafferty J, Griffiths AD, Winter G, Chiswell DJ. Phage antibodies: filamentous phage displaying antibody variable domains. Nature. 1990;348:552–4.
Smith GP. Filamentous fusion phage: novel expression vectors that display cloned antigens on the virion surface. Science. 1985;228:1315–7.
Wu CH, Liu IJ, Lu RM, Wu HC. Advancement and applications of peptide phage display technology in biomedical science. J Biomed Sci. 2016;23:8.
Article PubMed PubMed Central CAS Google Scholar
Kempeni J. Preliminary results of early clinical trials with the fully human anti-TNFalpha monoclonal antibody D2E7. Ann Rheum Dis. 1999;58(Suppl 1):I70–2.
Bartlett BL, Tyring SK. Ustekinumab for chronic plaque psoriasis. Lancet. 2008;371:1639–40.
Article PubMed Google Scholar
Church LD, McDermott MF. Canakinumab, a fully-human mAb against IL-1beta for the potential treatment of inflammatory disorders. Curr Opin Mol Ther. 2009;11:81–9.
CAS PubMed Google Scholar
Zhou H, Jang H, Fleischmann RM, Bouman-Thio E, Xu Z, Marini JC, et al. Pharmacokinetics and safety of golimumab, a fully human anti-TNF-alpha monoclonal antibody, in subjects with rheumatoid arthritis. J Clin Pharmacol. 2007;47:383–96.
Coiffier B, Lepretre S, Pedersen LM, Gadeberg O, Fredriksen H, van Oers MH, et al. Safety and efficacy of ofatumumab, a fully human monoclonal anti-CD20 antibody, in patients with relapsed or refractory B-cell chronic lymphocytic leukemia: a phase 1–2 study. Blood. 2008;111:1094–100.
Reddy GK, Nadler E, Jain VK. Denosumab (AMG 162), a Fully Human Monoclonal Antibody Against RANK Ligand Activity. Support Cancer Ther. 2005;3:14–5.
Morse MA. Technology evaluation: ipilimumab, Medarex/Bristol-Myers Squibb. Curr Opin Mol Ther. 2005;7:588–97.
Wolchok JD, Kluger H, Callahan MK, Postow MA, Rizvi NA, Lesokhin AM, et al. Nivolumab plus ipilimumab in advanced melanoma. N Engl J Med. 2013;369:122–33.
Roth EM, Diller P. Alirocumab for hyperlipidemia: physiology of PCSK9 inhibition, pharmacodynamics and Phase I and II clinical trial results of a PCSK9 monoclonal antibody. Futur Cardiol. 2014;10:183–99.
Article CAS Google Scholar
de Weers M, Tai YT, van der Veer MS, Bakker JM, Vink T, Jacobs DC, et al. Daratumumab, a novel therapeutic human CD38 monoclonal antibody, induces killing of multiple myeloma and other hematological tumors. J Immunol. 2011;186:1840–8.
Article PubMed CAS Google Scholar
Hirayama A, Honarpour N, Yoshida M, Yamashita S, Huang F, Wasserman SM, et al. Effects of evolocumab (AMG 145), a monoclonal antibody to PCSK9, in hypercholesterolemic, statin-treated Japanese patients at high cardiovascular risk--primary results from the phase 2 YUKAWA study. Circ J. 2014;78:1073–82.
Chioato A, Noseda E, Stevens M, Gaitatzis N, Kleinschmidt A, Picaud H. Treatment with the interleukin-17A-blocking antibody secukinumab does not interfere with the efficacy of influenza and meningococcal vaccinations in healthy subjects: results of an open-label, parallel-group, randomized single-center study. Clin Vacc Immunol: CVI. 2012;19:1597–602.
Chiorean EG, Sweeney C, Youssoufian H, Qin A, Dontabhaktuni A, Loizos N, et al. A phase I study of olaratumab, an anti-platelet-derived growth factor receptor alpha (PDGFRalpha) monoclonal antibody, in patients with advanced solid tumors. Cancer Chemother Pharmacol. 2014;73:595–604.
Papp KA, Leonardi C, Menter A, Ortonne JP, Krueger JG, Kricorian G, et al. Brodalumab, an anti-interleukin-17-receptor antibody for psoriasis. N Engl J Med. 2012;366:1181–9.
Wenzel S, Ford L, Pearlman D, Spector S, Sher L, Skobieranda F, et al. Dupilumab in persistent asthma with elevated eosinophil levels. N Engl J Med. 2013;368:2455–66.
Antonia S, Goldberg SB, Balmanoukian A, Chaft JE, Sanborn RE, Gupta A, et al. Safety and antitumour activity of durvalumab plus tremelimumab in non-small cell lung cancer: a multicentre, phase 1b study. Lancet Oncol. 2016;17:299–308.
Huizinga TW, Fleischmann RM, Jasson M, Radin AR, van Adelsberg J, Fiore S, et al. Sarilumab, a fully human monoclonal antibody against IL-6Ralpha in patients with rheumatoid arthritis and an inadequate response to methotrexate: efficacy and safety results from the randomised SARIL-RA-MOBILITY Part A trial. Ann Rheum Dis. 2014;73:1626–34.
Tepper S, Ashina M, Reuter U, Brandes JL, Dolezil D, Silberstein S, et al. Safety and efficacy of erenumab for preventive treatment of chronic migraine: a randomised, double-blind, placebo-controlled phase 2 trial. Lancet Neurol. 2017;16:425–34.
Migden MR, Rischin D, Schmults CD, Guminski A, Hauschild A, Lewis KD, et al. PD-1 Blockade with Cemiplimab in Advanced Cutaneous Squamous-Cell Carcinoma. N Engl J Med. 2018;379:341–51.
Lonberg N, Taylor LD, Harding FA, Trounstine M, Higgins KM, Schramm SR, et al. Antigen-specific human antibodies from mice comprising four distinct genetic modifications. Nature. 1994;368:856–9.
Mendez MJ, Green LL, Corvalan JR, Jia XC, Maynard-Currie CE, Yang XD, et al. Functional transplant of megabase human immunoglobulin loci recapitulates human antibody response in mice. Nat Genet. 1997;15:146–56.
Green LL, Hardy MC, Maynard-Currie CE, Tsuda H, Louie DM, Mendez MJ, et al. Antigen-specific human monoclonal antibodies from mice engineered with human Ig heavy and light chain YACs. Nat Genet. 1994;7:13–21.
Moroni M, Veronese S, Benvenuti S, Marrapese G, Sartore-Bianchi A, Di Nicolantonio F, et al. Gene copy number for epidermal growth factor receptor (EGFR) and clinical response to antiEGFR treatment in colorectal cancer: a cohort study. Lancet Oncol. 2005;6:279–86.
Gibson TB, Ranganathan A, Grothey A. Randomized phase III trial results of panitumumab, a fully human anti-epidermal growth factor receptor monoclonal antibody, in metastatic colorectal cancer. Clin Colorectal Cancer. 2006;6:29–31.
Labrijn AF, Janmaat ML, Reichert JM, Parren PWHI. Bispecific antibodies: a mechanistic review of the pipeline. Nat Rev Drug Discov. 2019;18:585–608.
Heiss MM, Murawa P, Koralewski P, Kutarska E, Kolesnik OO, Ivanchenko VV, et al. The trifunctional antibody catumaxomab for the treatment of malignant ascites due to epithelial cancer: Results of a prospective randomized phase II/III trial. Int J Cancer. 2010;127:2209–21.
Gokbuget N, Dombret H, Bonifacio M, Reichle A, Graux C, Faul C, et al. Blinatumomab for minimal residual disease in adults with B-cell precursor acute lymphoblastic leukemia. Blood. 2018;131:1522–31.
Oldenburg J, Mahlangu JN, Kim B, Schmitt C, Callaghan MU, Young G, et al. Emicizumab Prophylaxis in Hemophilia A with Inhibitors. N Engl J Med. 2017;377:809–18.
Kyowa Hakko Kirin Co. Ltd. Consolidated Financial Summary (IFRS) Fiscal 2018. 2019, February 5.
Grilo AL, Mantalaris A. The Increasingly Human and Profitable Monoclonal Antibody Market. Trends Biotechnol. 2019;37:9–16.
Donini C, D'Ambrosio L, Grignani G, Aglietta M, Sangiolo D. Next generation immune-checkpoints for cancer therapy. J Thorac Dis. 2018;10:S1581–S601.
Article PubMed PubMed Central Google Scholar
Sator P. Safety and tolerability of adalimumab for the treatment of psoriasis: a review summarizing 15 years of real-life experience. Ther Adv Chronic Dis. 2018;9:147–58.
Burmester GR, Panaccione R, Gordon KB, McIlraith MJ, Lacerda AP. Adalimumab: long-term safety in 23 458 patients from global clinical trials in rheumatoid arthritis, juvenile idiopathic arthritis, ankylosing spondylitis, psoriatic arthritis, psoriasis and Crohn's disease. Ann Rheum Dis. 2013;72:517–24.
Zamora-Atenza C, Diaz-Torne C, Geli C, Diaz-Lopez C, Ortiz MA, Moya P, et al. Adalimumab regulates intracellular TNFalpha production in patients with rheumatoid arthritis. Arthritis Res Ther. 2014;16:R153.
Pardoll DM. The blockade of immune checkpoints in cancer immunotherapy. Nat Rev Cancer. 2012;12:252–64.
Wang C, Thudium KB, Han M, Wang XT, Huang H, Feingersh D, et al. In vitro characterization of the anti-PD-1 antibody nivolumab, BMS-936558, and in vivo toxicology in non-human primates. Cancer Immunol Res. 2014;2:846–56.
Ansell SM, Lesokhin AM, Borrello I, Halwani A, Scott EC, Gutierrez M, et al. PD-1 blockade with nivolumab in relapsed or refractory Hodgkin's lymphoma. N Engl J Med. 2015;372:311–9.
Patnaik A, Kang SP, Rasco D, Papadopoulos KP, Elassaiss-Schaap J, Beeram M, et al. Phase I Study of Pembrolizumab (MK-3475; Anti-PD-1 Monoclonal Antibody) in Patients with Advanced Solid Tumors. Clin Cancer Res. 2015;21:4286–93.
Nghiem PT, Bhatia S, Lipson EJ, Kudchadkar RR, Miller NJ, Annamalai L, et al. PD-1 Blockade with Pembrolizumab in Advanced Merkel-Cell Carcinoma. N Engl J Med. 2016;374:2542–52.
Goldberg SB, Gettinger SN, Mahajan A, Chiang AC, Herbst RS, Sznol M, et al. Pembrolizumab for patients with melanoma or non-small-cell lung cancer and untreated brain metastases: early analysis of a non-randomised, open-label, phase 2 trial. Lancet Oncol. 2016;17:976–83.
Velcheti V, Chandwani S, Chen X, Pietanza MC, Burke T. First-line pembrolizumab monotherapy for metastatic PD-L1-positive NSCLC: real-world analysis of time on treatment. Immunotherapy. 2019;11:889–901.
Reck M, Rodriguez-Abreu D, Robinson AG, Hui R, Csoszi T, Fulop A, et al. Pembrolizumab versus Chemotherapy for PD-L1-Positive Non-Small-Cell Lung Cancer. N Engl J Med. 2016;375:1823–33.
Morgensztern D, Herbst RS. Nivolumab and Pembrolizumab for Non-Small Cell Lung Cancer. Clin Cancer Res. 2016;22:3713–7.
US Food and Drug Administration. FDA approves novel preventive treatment for migraine. 2018, May 17.
Paemeleire K, MaassenVanDenBrink A. Calcitonin-gene-related peptide pathway mAbs and migraine prevention. Curr Opin Neurol. 2018;31:274–80.
Liang YL, Khoshouei M, Deganutti G, Glukhova A, Koole C, Peat TS, et al. Cryo-EM structure of the active, Gs-protein complexed, human CGRP receptor. Nature. 2018;561:492–7.
Edvinsson L, Haanes KA, Warfvinge K, Krause DN. CGRP as the target of new migraine therapies - successful translation from bench to clinic. Nat Rev Neurol. 2018;14:338–50.
Migone TS, Subramanian GM, Zhong J, Healey LM, Corey A, Devalaraja M, et al. Raxibacumab for the treatment of inhalational anthrax. N Engl J Med. 2009;361:135–44.
Malley R, DeVincenzo J, Ramilo O, Dennehy PH, Meissner HC, Gruber WC, et al. Reduction of respiratory syncytial virus (RSV) in tracheal aspirates in intubated infants by use of humanized monoclonal antibody to RSV F protein. J Infect Dis. 1998;178:1555–61.
Emu B, Fessel J, Schrader S, Kumar P, Richmond G, Win S, et al. Phase 3 Study of Ibalizumab for Multidrug-Resistant HIV-1. N Engl J Med. 2018;379:645–54.
Sanofi EL. FDA to review isatuximab as a potential treatment for relapsed/refractory multiple myeloma. Paris, 2019.
Richardson PG, Attal M, Campana F, Le-Guennec S, Hui AM, Risse ML, et al. Isatuximab plus pomalidomide/dexamethasone versus pomalidomide/dexamethasone in relapsed/refractory multiple myeloma: ICARIA Phase III study design. Future Oncol. 2018;14:1035–47.
Novartis AG. Novartis Financial Results – Q2 2018. July 18, 2018 https://www.novartis.com/news/novartis-financial-results-q2-2018 Accessed 15 Jul 2019 .
TESARO Inc. TESARO Announces Data Presentations at ESMO 2018 Congress. Oct 20, 2018 https://www.globenewswire.com/news-release/2018/10/20/1624331/0/en/TESARO-Announces-Data-Presentations-at-ESMO-2018-Congress.html Accessed 15 Jul 2019.
TG Therapeutics I. TG Therapeutics announces update regarding UNITY-CLL Phase 3 trial. Sep 25, 3018 http://ir.tgtherapeutics.com/news-releases/news-release-details/tg-therapeutics-announces-update-regarding-unity-cll-phase-3 Accessed 15 Jul 2019.
Gorman SD, Clark MR. Humanisation of monoclonal antibodies for therapy. Semin Immunol. 1990;2:457–66.
Mountain A, Adair JR. Engineering antibodies for therapy. Biotechnol Genet Eng Rev. 1992;10:1–142.
Queen C, Schneider WP, Selick HE, Payne PW, Landolfi NF, Duncan JF, et al. A humanized antibody that binds to the interleukin 2 receptor. Proc Natl Acad Sci U S A. 1989;86:10029–33.
Choi Y, Hua C, Sentman CL, Ackerman ME, Bailey-Kellogg C. Antibody humanization by structure-based computational protein design. MAbs. 2015;7:1045–57.
Olimpieri PP, Marcatili P, Tramontano A. Tabhu: tools for antibody humanization. Bioinformatics. 2015;31:434–5.
Swindells MB, Porter CT, Couch M, Hurst J, Abhinandan K, Nielsen JH, et al. abYsis: integrated antibody sequence and structure—management, analysis, and prediction. J Mol Biol. 2017;429:356–64.
Abhinandan KR, Martin ACR. Analyzing the “Degree of Humanness” of Antibody Sequences. J Mol Biol. 2007;369:852–62.
Pelat T, Bedouelle H, Rees AR, Crennell SJ, Lefranc M-P, Thullier P. Germline Humanization of a Non-human Primate Antibody that Neutralizes the Anthrax Toxin, by in Vitro and in Silico Engineering. J Mol Biol. 2008;384:1400–7.
Thullier P, Huish O, Pelat T, Martin ACR. The Humanness of Macaque Antibody Sequences. J Mol Biol. 2010;396:1439–50.
Gao SH, Huang K, Tu H, Adler AS. Monoclonal antibody humanness score and its applications. BMC Biotechnol. 2013;13:55.
Ducancel F, Muller BH. Molecular engineering of antibodies for therapeutic and diagnostic purposes. MAbs. 2012;4:445–57.
Harding FA, Stickler MM, Razo J, DuBridge RB. The immunogenicity of humanized and fully human antibodies: residual immunogenicity resides in the CDR regions. MAbs. 2010;2:256–65.
Hansel TT, Kropshofer H, Singer T, Mitchell JA, George AJ. The safety and side effects of monoclonal antibodies. Nat Rev Drug Discov. 2010;9:325.
Waldmann H. Human Monoclonal Antibodies: The Benefits of Humanization. Methods Mol Biol. 1904;2019:1–10.
Google Scholar
Rebello PRUB, Hale G, Friend PJ, Cobbold SP, Waldmann H. Anti-globulin responses to rat and humanized campath-1 monoclonal antibody used to treat transplant rejection1. Transplantation. 1999;68:1417–9.
Cobleigh MA, Vogel CL, Tripathy D, Robert NJ, Scholl S, Fehrenbacher L, et al. Multinational study of the efficacy and safety of humanized anti-HER2 monoclonal antibody in women who have HER2-overexpressing metastatic breast cancer that has progressed after chemotherapy for metastatic disease. J Clin Oncol. 1999;17:2639–48.
Jackisch C, Kim SB, Semiglazov V, Melichar B, Pivot X, Hillenbach C, et al. Subcutaneous versus intravenous formulation of trastuzumab for HER2-positive early breast cancer: updated results from the phase III HannaH study. Ann Oncol. 2015;26:320–5.
Harding FA, Stickler MM, Razo J, DuBridge RB. The immunogenicity of humanized and fully human antibodies: residual immunogenicity resides in the CDR regions. mAbs. 2010;2:256–65.
Bender NK, Heilig CE, Dröll B, Wohlgemuth J, Armbruster F-P, Heilig B. Immunogenicity, efficacy and adverse events of adalimumab in RA patients. Rheumatol Int. 2007;27:269–74.
West RL, Zelinkova Z, Wolbink GJ, Kuipers EJ, Stokkers PC, van der Woude CJ. Immunogenicity negatively influences the outcome of adalimumab treatment in Crohn's disease. Aliment Pharmacol Ther. 2008;28:1122–6.
Kay J, Matteson EL, Dasgupta B, Nash P, Durez P, Hall S, et al. Golimumab in patients with active rheumatoid arthritis despite treatment with methotrexate: a randomized, double-blind, placebo-controlled, dose-ranging study. Arthritis Rheum. 2008;58:964–75.
Parmley SF, Smith GP. Antibody-selectable filamentous fd phage vectors: affinity purification of target genes. Gene. 1988;73:305–18.
Clackson T, Hoogenboom HR, Griffiths AD, Winter G. Making antibody fragments using phage display libraries. Nature. 1991;352:624–8.
Vaughan TJ, Williams AJ, Pritchard K, Osbourn JK, Pope AR, Earnshaw JC, et al. Human antibodies with sub-nanomolar affinities isolated from a large non-immunized phage display library. Nat Biotechnol. 1996;14:309–14.
Breitling F, Dubel S, Seehaus T, Klewinghaus I, Little M. A surface expression vector for antibody screening. Gene. 1991;104:147–53.
Barbas CF, Kang AS, Lerner RA, Benkovic SJ. Assembly of combinatorial antibody libraries on phage surfaces: the gene III site. Proc Natl Acad Sci U S A. 1991;88:7978–82.
Nixon AE, Sexton DJ, Ladner RC. Drugs derived from phage display: from candidate identification to clinical practice. MAbs. 2014;6:73–85.
Sheets MD, Amersdorfer P, Finnern R, Sargent P, Lindqvist E, Schier R, et al. Efficient construction of a large nonimmune phage antibody library: The production of high-affinity human single-chain antibodies to protein antigens. Proc Natl Acad Sci U S A. 1998;95:6157–62.
Lu RM, Chang YL, Chen MS, Wu HC. Single chain anti-c-Met antibody conjugated nanoparticles for in vivo tumor-targeted imaging and drug delivery. Biomaterials. 2011;32:3265–74.
Bradbury ARM, Sidhu S, Dübel S, McCafferty J. Beyond natural antibodies: the power of in vitro display technologies. Nat Biotechnol. 2011;29:245–54.
Lerner RA. Combinatorial antibody libraries: new advances, new immunological insights. Nat Rev Immunol. 2016;16:498–508.
Weber M, Bujak E, Putelli A, Villa A, Matasci M, Gualandi L, et al. A highly functional synthetic phage display library containing over 40 billion human antibody clones. PLoS One. 2014;9:e100000.
Lee TY, Wu HC, Tsao TC and Lin W; Fountain Biopharma Inc., assignee. Antibodies to interleukin-6 US patent US 9,234,035. 2016 January 12.
Wu HC, Lu RM, Chiu CY, Liu IJ and Chang YL; Academia Sinica, assignee. Anti-vascular endothelial growth factor receptor 2 (VEGFR2) antibody and methods of use thereof for detecting VEGFR2 and for inhibiting tumor growth, tumor angiogenesis and/or inducing cancer cell cytotoxicity. US patent US10,196,447. 2018 February 5.
de Haard HJ, van Neer N, Reurs A, Hufton SE, Roovers RC, Henderikx P, et al. A large non-immunized human Fab fragment phage library that permits rapid isolation and kinetic analysis of high affinity antibodies. J Biol Chem. 1999;274:18218–30.
Knappik A, Ge L, Honegger A, Pack P, Fischer M, Wellnhofer G, et al. Fully synthetic human combinatorial antibody libraries (HuCAL) based on modular consensus frameworks and CDRs randomized with trinucleotides. J Mol Biol. 2000;296:57–86.
Rothe C, Urlinger S, Lohning C, Prassler J, Stark Y, Jager U, et al. The human combinatorial antibody library HuCAL GOLD combines diversification of all six CDRs according to the natural immune system with a novel display method for efficient selection of high-affinity antibodies. J Mol Biol. 2008;376:1182–200.
Chan CE, Lim AP, MacAry PA, Hanson BJ. The role of phage display in therapeutic antibody discovery. Int Immunol. 2014;26:649–57.
Chan CE, Chan AH, Lim AP, Hanson BJ. Comparison of the efficiency of antibody selection from semi-synthetic scFv and non-immune Fab phage display libraries against protein targets for rapid development of diagnostic immunoassays. J Immunol Methods. 2011;373:79–88.
Wrublewski DT. Analysis for Science Librarians of the 2018 Nobel Prize in Chemistry: Directed Evolution of Enzymes and Phage Display of Peptides and Antibodies. Sci Technol Libr. 2019:1–19.
Batlle E, Clevers H. Cancer stem cells revisited. Nat Med. 2017;23:1124.
Swaminathan SK, Niu L, Waldron N, Kalscheuer S, Zellmer DM, Olin MR, et al. Identification and characterization of a novel scFv recognizing human and mouse CD133. Drug Deliv Transl Res. 2013;3:143–51.
Nilvebrant J, Kuku G, Bjorkelund H, Nestor M. Selection and in vitro characterization of human CD44v6-binding antibody fragments. Biotechnol Appl Biochem. 2012;59:367–80.
Baskar S, Suschak JM, Samija I, Srinivasan R, Childs RW, Pavletic SZ, et al. A human monoclonal antibody drug and target discovery platform for B-cell chronic lymphocytic leukemia based on allogeneic hematopoietic stem cell transplantation and phage display. Blood. 2009;114:4494–502.
Zhu X, Bidlingmaier S, Hashizume R, James CD, Berger MS, Liu B. Identification of internalizing human single-chain antibodies targeting brain tumor sphere cells. Mol Cancer Ther. 2010;9:2131–41.
Larsen SA, Meldgaard T, Fridriksdottir AJ, Lykkemark S, Poulsen PC, Overgaard LF, et al. Selection of a breast cancer subpopulation-specific antibody using phage display on tissue sections. Immunol Res. 2015;62:263–72.
Sun Y, Shukla GS, Weaver D, Pero SC, Krag DN. Phage-display selection on tumor histological specimens with laser capture microdissection. J Immunol Methods. 2009;347:46–53.
Larsen SA, Meldgaard T, Lykkemark S, Mandrup OA, Kristensen P. Selection of cell-type specific antibodies on tissue-sections using phage display. J Cell Mol Med. 2015.
Su Y, Bidlingmaier S, Lee N-K, Liu B. Combine phage antibody display library selection on patient tissue specimens with laser capture microdissection to identify novel human antibodies targeting clinically relevant tumor antigens. In: Hust M, Lim TS, editors. Phage Display: Methods and Protocols. New York: Springer New York; 2018. p. 331–47.
Weiner GJ. Building better monoclonal antibody-based therapeutics. Nat Rev Cancer. 2015;15:361–70.
Rosenblum D, Joshi N, Tao W, Karp JM, Peer D. Progress and challenges towards targeted delivery of cancer therapeutics. Nat Commun. 2018;9:1410.
Caracciolo G. Clinically approved liposomal nanomedicines: lessons learned from the biomolecular corona. Nanoscale. 2018;10:4167–72.
Becerril B, Poul MA, Marks JD. Toward selection of internalizing antibodies from phage libraries. Biochem Biophys Res Commun. 1999;255:386–93.
Roth A, Drummond DC, Conrad F, Hayes ME, Kirpotin DB, Benz CC, et al. Anti-CD166 single chain antibody-mediated intracellular delivery of liposomal drugs to prostate cancer cells. Mol Cancer Ther. 2007;6:2737–46.
Maruyama T, Parren PW, Sanchez A, Rensink I, Rodriguez LL, Khan AS, et al. Recombinant human monoclonal antibodies to Ebola virus. J Infect Dis. 1999;179(Suppl 1):S235–9.
Flego M, Di Bonito P, Ascione A, Zamboni S, Carattoli A, Grasso F, et al. Generation of human antibody fragments recognizing distinct epitopes of the nucleocapsid (N) SARS-CoV protein using a phage display approach. BMC Infect Dis. 2005;5:73.
Kang X, Yang BA, Hu Y, Zhao H, Xiong W, Yang Y, et al. Human neutralizing Fab molecules against severe acute respiratory syndrome coronavirus generated by phage display. Clin Vacc Immunol: CVI. 2006;13:953–7.
Marasco WA, Sui J. The growth and potential of human antiviral monoclonal antibody therapeutics. Nat Biotechnol. 2007;25:1421–34.
Lerner RA. Manufacturing Immunity to Disease in a Test Tube: The Magic Bullet Realized. Angew Chem Int Ed. 2006;45:8106–25.
Zhang X, Qi X, Zhang Q, Zeng X, Shi Z, Jin Q, et al. Human 4F5 single-chain Fv antibody recognizing a conserved HA1 epitope has broad neutralizing potency against H5N1 influenza A viruses of different clades. Antivir Res 2013;99:91–9.
Kashyap AK, Steel J, Oner AF, Dillon MA, Swale RE, Wall KM, et al. Combinatorial antibody libraries from survivors of the Turkish H5N1 avian influenza outbreak reveal virus neutralization strategies. Proc Natl Acad Sci U S A. 2008;105:5986–91.
Chen Z, Wang J, Bao L, Guo L, Zhang W, Xue Y, et al. Human monoclonal antibodies targeting the haemagglutinin glycoprotein can neutralize H7N9 influenza virus. Nat Commun. 2015;6.
Wang J, Chen Z, Bao L, Zhang W, Xue Y, Pang X, et al. Characterization of Two Human Monoclonal Antibodies Neutralizing Influenza A H7N9 Viruses. J Virol. 2015.
Cotten M, Watson SJ, Zumla AI, Makhdoom HQ, Palser AL, Ong SH, et al. Spread, circulation, and evolution of the Middle East respiratory syndrome coronavirus. mBio. 2014;5.
Tang XC, Agnihothram SS, Jiao Y, Stanhope J, Graham RL, Peterson EC, et al. Identification of human neutralizing antibodies against MERS-CoV and their role in virus adaptive evolution. Proc Natl Acad Sci U S A. 2014;111:E2018–E26.
Lim CC, Woo PCY, Lim TS. Development of a Phage Display Panning Strategy Utilizing Crude Antigens: Isolation of MERS-CoV Nucleoprotein human antibodies. Sci Rep. 2019;9:6088.
Walker LM, Burton DR. Passive immunotherapy of viral infections: 'super-antibodies' enter the fray. Nat Rev Immunol. 2018;18:297–308.
Kennedy PJ, Oliveira C, Granja PL, Sarmento B. Monoclonal antibodies: technologies for early discovery and engineering. Crit Rev Biotechnol. 2018;38:394–408.
Weinblatt ME, Keystone EC, Furst DE, Moreland LW, Weisman MH, Birbara CA, et al. Adalimumab, a fully human anti-tumor necrosis factor alpha monoclonal antibody, for the treatment of rheumatoid arthritis in patients taking concomitant methotrexate: the ARMADA trial. Arthritis Rheum. 2003;48:35–45.
Lindsley CW. Predictions and Statistics for the Best-Selling Drugs Globally and in the United States in 2018 and a Look Forward to 2024 Projections. ACS Chem Neurosci. 2019;10:1115.
Edwards BM, Barash SC, Main SH, Choi GH, Minter R, Ullrich S, et al. The remarkable flexibility of the human antibody repertoire; isolation of over one thousand different antibodies to a single protein, BLyS. J Mol Biol. 2003;334:103–18.
Sanz I, Yasothan U, Kirkpatrick P. Belimumab. Nat Rev Drug Discov. 2011;10:335.
Dimitrov DS, Marks JD. Therapeutic antibodies: current state and future trends--is a paradigm change coming soon? Methods Mol Biol. 2009;525:1–27 xiii.
Diaz-Serrano A, Sanchez-Torre A, Paz-Ares L. Necitumumab for the treatment of advanced non-small-cell lung cancer. Future Oncol. 2019;15:705–16.
Arrieta O, Zatarain-Barron ZL, Cardona AF, Carmona A, Lopez-Mejia M. Ramucirumab in the treatment of non-small cell lung cancer. Expert Opin Drug Saf. 2017;16:637–44.
Aprile G, Ferrari L, Cremolini C, Bergamo F, Fontanella C, Battaglin F, et al. Ramucirumab for the treatment of gastric cancers, colorectal adenocarcinomas, and other gastrointestinal malignancies. Expert Rev Clin Pharmacol. 2016;9:877–85.
Lu D, Jimenez X, Zhang H, Bohlen P, Witte L, Zhu Z. Selection of high affinity human neutralizing antibodies to VEGFR2 from a large antibody phage display library for antiangiogenesis therapy. Int J Cancer. 2002;97:393–9.
Lu D, Shen J, Vil MD, Zhang H, Jimenez X, Bohlen P, et al. Tailoring in vitro selection for a picomolar affinity human antibody directed against vascular endothelial growth factor receptor 2 for enhanced neutralizing activity. J Biol Chem. 2003;278:43496–507.
Frenzel A, Schirrmann T, Hust M. Phage display-derived human antibodies in clinical development and therapy. MAbs. 2016;8:1177–94.
Boyerinas B, Jochems C, Fantini M, Heery CR, Gulley JL, Tsang KY, et al. Antibody-Dependent Cellular Cytotoxicity Activity of a Novel Anti–PD-L1 Antibody Avelumab (MSB0010718C) on Human Tumor Cells. Cancer Immunol Res. 2015.
Rodriguez-Vida A, Bellmunt J. Avelumab for the treatment of urothelial cancer. Expert Rev Anticancer Ther. 2018;18:421–9.
Benson J, Cunningham M, Duchala C, Giles-Komar JM, Luo J, Rycyzyn MA, et al.; Janssen Biotech Inc., assignee. Anti-IL-23 antibodies, compositions, methods and uses. US patent US7807414B2. 2009.
Benson J, Carton J, Cunningham M, Orlovsky YI, Rauchenberger R and Sweet R; Janssen Biotech Inc., assignee. Anti-IL-23 antibody compositions. US patent US9714287B2. 2016.
Machado A, Torres T. Guselkumab for the Treatment of Psoriasis. Biodrugs. 2018;32:119–28.
Bork K, Meng G, Staubach P, Hardt J. Hereditary angioedema: new findings concerning symptoms, affected organs, and course. Am J Med. 2006;119:267–74.
Cicardi M, Banerji A, Bracho F, Malbrán A, Rosenkranz B, Riedl M, et al. Icatibant, a new bradykinin-receptor antagonist, in hereditary angioedema. N Engl J Med. 2010;363:532–41.
Busse PJ, Farkas H, Banerji A, Lumry WR, Longhurst HJ, Sexton DJ, et al. Lanadelumab for the Prophylactic Treatment of Hereditary Angioedema with C1 Inhibitor Deficiency: A Review of Preclinical and Phase I Studies. BioDrugs. 2019;33:33–43.
Xu C, Sui J, Tao H, Zhu Q, Marasco WA. Human anti-CXCR4 antibodies undergo VH replacement, exhibit functional V-region sulfation, and define CXCR4 antigenic heterogeneity. J Immunol. 2007;179:2408–18.
Sheehan J and Marasco WA. Phage and Yeast Display. Microbiol Spectr. 2015;3:Aid-0028-2014.
Bradbury AR, Marks JD. Antibodies from phage antibody libraries. J Immunol Methods. 2004;290:29–49.
Jones AR, Stutz CC, Zhou Y, Marks JD, Shusta EV. Identifying blood-brain-barrier selective single-chain antibody fragments. Biotechnol J. 2014;9:664–74.
Bruggemann M, Osborn MJ, Ma B, Hayre J, Avis S, Lundstrom B, et al. Human antibody production in transgenic animals. Arch Immunol Ther Exp (Warsz). 2015;63:101–8.
Osborn MJ, Ma B, Avis S, Binnie A, Dilley J, Yang X, et al. High-affinity IgG antibodies develop naturally in Ig-knockout rats carrying germline human IgH/Igkappa/Iglambda loci bearing the rat CH region. J Immunol. 2013;190:1481–90.
Lee EC, Liang Q, Ali H, Bayliss L, Beasley A, Bloomfield-Gerdes T, et al. Complete humanization of the mouse immunoglobulin loci enables efficient therapeutic antibody discovery. Nat Biotechnol. 2014;32:356–63.
Murphy AJ, Macdonald LE, Stevens S, Karow M, Dore AT, Pobursky K, et al. Mice with megabase humanization of their immunoglobulin genes generate antibodies as efficiently as normal mice. Proc Natl Acad Sci U S A. 2014;111:5153–8.
Alt FW, Keith Blackwell T, Yancopoulos GD. Immunoglobulin genes in transgenic mice. Trends Genet. 1985;1:231–6.
Bruggemann M, Caskey HM, Teale C, Waldmann H, Williams GT, Surani MA, et al. A repertoire of monoclonal antibodies with human heavy chains from transgenic mice. Proc Natl Acad Sci U S A. 1989;86:6709–13.
Taylor LD, Carmack CE, Schramm SR, Mashayekh R, Higgins KM, Kuo CC, et al. A transgenic mouse that expresses a diversity of human sequence heavy and light chain immunoglobulins. Nucleic Acids Res. 1992;20:6287–95.
Chen J, Trounstine M, Alt FW, Young F, Kurahara C, Loring JF, et al. Immunoglobulin gene rearrangement in B cell deficient mice generated by targeted deletion of the JH locus. Int Immunol. 1993;5:647–56.
Chen J, Trounstine M, Kurahara C, Young F, Kuo CC, Xu Y, et al. B cell development in mice that lack one or both immunoglobulin kappa light chain genes. EMBO J. 1993;12:821–30.
Davies NP, Rosewell IR, Richardson JC, Cook GP, Neuberger MS, Brownstein BH, et al. Creation of mice expressing human antibody light chains by introduction of a yeast artificial chromosome containing the core region of the human immunoglobulin kappa locus. Biotechnology (N Y). 1993;11:911–4.
CAS Google Scholar
Choi TK, Hollenbach PW, Pearson BE, Ueda RM, Weddell GN, Kurahara CG, et al. Transgenic mice containing a human heavy chain immunoglobulin gene fragment cloned in a yeast artificial chromosome. Nat Genet. 1993;4:117–23.
Jakobovits A, Amado RG, Yang X, Roskos L, Schwab G. From XenoMouse technology to panitumumab, the first fully human antibody product from transgenic mice. Nat Biotechnol. 2007;25:1134–43.
Hoffman W, Lakkis FG, Chalasani G. B Cells, Antibodies, and More. Clin J Am Soc Nephrol. 2016;11:137–54.
Diaz M, Casali P. Somatic immunoglobulin hypermutation. Curr Opin Immunol. 2002;14:235–40.
Nimmerjahn F, Ravetch JV. Fc-receptors as regulators of immunity. Adv Immunol. 2007;96:179–204.
Tyagi P. Recent results and ongoing trials with panitumumab (ABX-EGF), a fully human anti-epidermal growth factor receptor antibody, in metastatic colorectal cancer. Clin Colorectal Cancer. 2005;5:21–3.
Den Broeder, Alfons, et al. A single dose, placebo controlled study of the fully human anti-tumor necrosis factor-alpha antibody adalimumab (D2E7) in patients with rheumatoid arthritis. J Rheumatol. 2002;29(11):2288–98.
Tyagi, Preeta, Chu E, Jain VK. Recent results and ongoing trials with panitumumab (ABXEGF), a fully human anti–epidermal growth factor receptor antibody, in metastatic colorectal cancer. Clin Colorectal Cancer. 2005;5(1):21–23.
Ding, Changhai. Belimumab, an anti-BLyS human monoclonal antibody for potential treatment of inflammatory autoimmune diseases. Expert Opin Biol Ther. 2008;8(11):1805–14.
Krupitskaya, Yelena, Wakelee HA. Ramucirumab, a fully human mAb to the transmembrane signaling tyrosine kinase VEGFR-2 for the potential treatment of cancer. Curr Opin Investig Drugs. 2009;10(6):597–605.
Kuenen, Bart, et al. A phase I pharmacologic study of necitumumab (IMC-11F8), a fully human IgG1 monoclonal antibody directed against EGFR in patients with advanced solid malignancies. Clin Cancer Res. 2010;16(6):1915–23.
McDermott, David F, et al. Atezolizumab, an anti–programmed death-ligand 1 antibody, in metastatic renal cell carcinoma: long-term safety, clinical activity, and immune correlates from a phase Ia study. J Clin Oncol. 2016;34(8):833–42.
Boyerinas, Benjamin, et al. Antibody-dependent cellular cytotoxicity activity of a novel anti–PD-L1 antibody avelumab (MSB0010718C) on human tumor cells. Cancer Immun Res. 2015;3(10):1148–57.
Sofen, Howard, et al. Guselkumab (an IL-23–specific mAb) demonstrates clinical and molecular response in patients with moderate-to-severe psoriasis. J Allergy Clin Immunol. 2014;133(4):1032–40.
Al-Salama, Zaina T. Emapalumab: first global approval. Drugs. 2019;79(1):99–103.
Kreitman, Robert J, et al. Moxetumomab pasudotox in relapsed/refractory hairy cell leukemia. Leukemia. 2018;32(8):1768.
Berardi R, Onofri A, Pistelli M, Maccaroni E, Scartozzi M, Pierantoni C, et al. Panitumumab: the evidence for its use in the treatment of metastatic colorectal cancer. Core Evid. 2010;5:61–76.
Wong IT, Shojania K, Dutz J, Tsao NW. Clinical and economic review of secukinumab for moderate-to-severe plaque psoriasis. Expert Rev Pharmacoecon Outcomes Res. 2016;16:153–66.
Gibney GT, Hamid O, Lutzky J, Olszanski AJ, Mitchell TC, Gajewski TF, et al. Phase 1/2 study of epacadostat in combination with ipilimumab in patients with unresectable or metastatic melanoma. J Immunother Cancer. 2019;7:80.
Brahmer JR, Hammers H, Lipson EJ. Nivolumab: targeting PD-1 to bolster antitumor immunity. Future Oncol. 2015;11:1307–26.
Adedokun OJ, Xu Z, Gasink C, Jacobstein D, Szapary P, Johanns J, et al. Pharmacokinetics and Exposure Response Relationships of Ustekinumab in Patients With Crohn's Disease. Gastroenterology. 2018;154:1660–71.
Scott CT. Mice with a human touch. Nat Biotechnol. 2007;25:1075–7.
Legouffe E, Liautard J, Gaillard JP, Rossi JF, Wijdenes J, Bataille R, et al. Human anti-mouse antibody response to the injection of murine monoclonal antibodies against IL-6. Clin Exp Immunol. 1994;98:323–9.
Aman P, Ehlin-Henriksson B, Klein G. Epstein-Barr virus susceptibility of normal human B lymphocyte populations. J Exp Med. 1984;159:208–20.
Traggiai E, Becker S, Subbarao K, Kolesnikova L, Uematsu Y, Gismondo MR, et al. An efficient method to make human monoclonal antibodies from memory B cells: potent neutralization of SARS coronavirus. Nat Med. 2004;10:871–5.
Bonsignori M, Hwang KK, Chen X, Tsao CY, Morris L, Gray E, et al. Analysis of a clonal lineage of HIV-1 envelope V2/V3 conformational epitope-specific broadly neutralizing antibodies and their inferred unmutated common ancestors. J Virol. 2011;85:9998–10009.
Kuppers R, Zhao M, Hansmann ML, Rajewsky K. Tracing B cell development in human germinal centres by molecular analysis of single cells picked from histological sections. EMBO J. 1993;12:4955–67.
Smith K, Garman L, Wrammert J, Zheng NY, Capra JD, Ahmed R, et al. Rapid generation of fully human monoclonal antibodies specific to a vaccinating antigen. Nat Protoc. 2009;4:372–84.
Obiakor H, Sehgal D, Dasso JF, Bonner RF, Malekafzali A, Mage RG. A comparison of hydraulic and laser capture microdissection methods for collection of single B cells, PCR, and sequencing of antibody VDJ. Anal Biochem. 2002;306:55–62.
Tiller T, Meffre E, Yurasov S, Tsuiji M, Nussenzweig MC, Wardemann H. Efficient generation of monoclonal antibodies from single human B cells by single cell RT-PCR and expression vector cloning. J Immunol Methods. 2008;329:112–24.
Wardemann H, Yurasov S, Schaefer A, Young JW, Meffre E, Nussenzweig MC. Predominant autoantibody production by early human B cell precursors. Science. 2003;301:1374–7.
Lagerkvist AC, Furebring C, Borrebaeck CA. Single, antigen-specific B cells used to generate Fab fragments using CD40-mediated amplification or direct PCR cloning. Biotechniques. 1995;18:862–9.
Battye FL, Light A, Tarlinton DM. Single cell sorting and cloning. J Immunol Methods. 2000;243:25–32.
Scheid JF, Mouquet H, Feldhahn N, Walker BD, Pereyra F, Cutrell E, et al. A method for identification of HIV gp140 binding memory B cells in human blood. J Immunol Methods. 2009;343:65–7.
Herzenberg LA, Parks D, Sahaf B, Perez O, Roederer M, Herzenberg LA. The history and future of the fluorescence activated cell sorter and flow cytometry: a view from Stanford. Clin Chem. 2002;48:1819–27.
Lundkvist A, Horling J, Athlin L, Rosen A, Niklasson B. Neutralizing human monoclonal antibodies against Puumala virus, causative agent of nephropathia epidemica: a novel method using antigen-coated magnetic beads for specific B cell isolation. J Gen Virol. 1993;74(Pt 7):1303–10.
Correa I, Ilieva KM, Crescioli S, Lombardi S, Figini M, Cheung A, et al. Evaluation of Antigen-Conjugated Fluorescent Beads to Identify Antigen-Specific B Cells. Front Immunol. 2018;9:493.
Weitkamp JH, Kallewaard N, Kusuhara K, Feigelstock D, Feng N, Greenberg HB, et al. Generation of recombinant human monoclonal antibodies to rotavirus from single antigen-specific B cells selected with fluorescent virus-like particles. J Immunol Methods. 2003;275:223–37.
Wu X, Yang ZY, Li Y, Hogerkorp CM, Schief WR, Seaman MS, et al. Rational design of envelope identifies broadly neutralizing human monoclonal antibodies to HIV-1. Science. 2010;329:856–61.
Scheid JF, Mouquet H, Feldhahn N, Seaman MS, Velinzon K, Pietzsch J, et al. Broad diversity of neutralizing antibodies isolated from memory B cells in HIV-infected individuals. Nature. 2009;458:636–40.
Smith SA, de Alwis AR, Kose N, Jadi RS, de Silva AM, Crowe JE Jr. Isolation of dengue virus-specific memory B cells with live virus antigen from human subjects following natural infection reveals the presence of diverse novel functional groups of antibody clones. J Virol. 2014;88:12233–41.
Woda M, Friberg H, Currier JR, Srikiatkhachorn A, Macareo LR, Green S, et al. Dynamics of Dengue Virus (DENV)-Specific B Cells in the Response to DENV Serotype 1 Infections, Using Flow Cytometry With Labeled Virions. J Infect Dis. 2016;214:1001–9.
Coronella JA, Telleman P, Truong TD, Ylera F, Junghans RP. Amplification of IgG VH and VL (Fab) from single human plasma cells and B cells. Nucleic Acids Res. 2000;28:E85.
Wrammert J, Smith K, Miller J, Langley WA, Kokko K, Larsen C, et al. Rapid cloning of high-affinity human monoclonal antibodies against influenza virus. Nature. 2008;453:667–71.
Jin A, Ozawa T, Tajiri K, Obata T, Kondo S, Kinoshita K, et al. A rapid and efficient single-cell manipulation method for screening antigen-specific antibody-secreting cells from human peripheral blood. Nat Med. 2009;15:1088–92.
Love JC, Ronan JL, Grotenbreg GM, van der Veen AG, Ploegh HL. A microengraving method for rapid selection of single cells producing antigen-specific antibodies. Nat Biotechnol. 2006;24:703–7.
Ogunniyi AO, Story CM, Papa E, Guillen E, Love JC. Screening individual hybridomas by microengraving to discover monoclonal antibodies. Nat Protoc. 2009;4:767–82.
Tajiri K, Kishi H, Tokimitsu Y, Kondo S, Ozawa T, Kinoshita K, et al. Cell-microarray analysis of antigen-specific B-cells: single cell analysis of antigen receptor expression and specificity. Cytometry A. 2007;71:961–7.
Tokimitsu Y, Kishi H, Kondo S, Honda R, Tajiri K, Motoki K, et al. Single lymphocyte analysis with a microwell array chip. Cytometry A. 2007;71:1003–10.
Smith K, Crowe SR, Garman L, Guthridge CJ, Muther JJ, McKee E, et al. Human monoclonal antibodies generated following vaccination with AVA provide neutralization by blocking furin cleavage but not by preventing oligomerization. Vaccine. 2012;30:4276–83.
Chi X, Li J, Liu W, Wang X, Yin K, Liu J, et al. Generation and Characterization of Human Monoclonal Antibodies Targeting Anthrax Protective Antigen following Vaccination with a Recombinant Protective Antigen Vaccine. Clin Vacc Immunol: CVI. 2015;22:553–60.
Rudkin FM, Raziunaite I, Workman H, Essono S, Belmonte R, MacCallum DM, et al. Single human B cell-derived monoclonal anti-Candida antibodies enhance phagocytosis and protect against disseminated candidiasis. Nat Commun. 2018;9:5288.
Cox KS, Tang A, Chen Z, Horton MS, Yan H, Wang XM, et al. Rapid isolation of dengue-neutralizing antibodies from single cell-sorted human antigen-specific memory B-cell cultures. MAbs. 2016;8:129–40.
Iizuka A, Komiyama M, Tai S, Oshita C, Kurusu A, Kume A, et al. Identification of cytomegalovirus (CMV)pp65 antigen-specific human monoclonal antibodies using single B cell-based antibody gene cloning from melanoma patients. Immunol Lett. 2011;135:64–73.
Tian C, Luskin GK, Dischert KM, Higginbotham JN, Shepherd BE, Crowe JE Jr. Immunodominance of the VH1–46 antibody gene segment in the primary repertoire of human rotavirus-specific B cells is reduced in the memory compartment through somatic mutation of nondominant clones. J Immunol. 2008;180:3279–88.
Di Niro R, Mesin L, Raki M, Zheng NY, Lund-Johansen F, Lundin KE, et al. Rapid generation of rotavirus-specific human monoclonal antibodies from small-intestinal mucosa. J Immunol. 2010;185:5377–83.
Bailey MJ, Duehr J, Dulin H, Broecker F, Brown JA, Arumemi FO, et al. Human antibodies targeting Zika virus NS1 provide protection against disease in a mouse model. Nat Commun. 2018;9:4560.
Bushey RT, Moody MA, Nicely NL, Haynes BF, Alam SM, Keir ST, et al. A Therapeutic Antibody for Cancer, Derived from Single Human B Cells. Cell Rep. 2016;15:1505–13.
Corti D, Misasi J, Mulangu S, Stanley DA, Kanekiyo M, Wollen S, et al. Protective monotherapy against lethal Ebola virus infection by a potently neutralizing antibody. Science. 2016;351:1339–42.
Rijal P, Elias SC, Machado SR, Xiao J, Schimanski L, O'Dowd V, et al. Therapeutic Monoclonal Antibodies for Ebola Virus Infection Derived from Vaccinated Humans. Cell Rep. 2019;27:172–86 e7.
Bornholdt ZA, Turner HL, Murin CD, Li W, Sok D, Souders CA, et al. Isolation of potent neutralizing antibodies from a survivor of the 2014 Ebola virus outbreak. Science. 2016;351:1078–83.
Awi NJ, Teow SY. Antibody-Mediated Therapy against HIV/AIDS: Where Are We Standing Now? J Pathog. 2018;2018:8724549.
Nogales A, Piepenbrink MS, Wang J, Ortega S, Basu M, Fucile CF, et al. A Highly Potent and Broadly Neutralizing H1 Influenza-Specific Human Monoclonal Antibody. Sci Rep. 2018;8:4374.
Fu Y, Zhang Z, Sheehan J, Avnir Y, Ridenour C, Sachnik T, et al. A broadly neutralizing anti-influenza antibody reveals ongoing capacity of haemagglutinin-specific memory B cells to evolve. Nat Commun. 2016;7:12780.
Nakamura G, Chai N, Park S, Chiang N, Lin Z, Chiu H, et al. An in vivo human-plasmablast enrichment technique allows rapid identification of therapeutic influenza A antibodies. Cell Host Microbe. 2013;14:93–103.
Chai N, Swem LR, Park S, Nakamura G, Chiang N, Estevez A, et al. A broadly protective therapeutic antibody against influenza B virus with two mechanisms of action. Nat Commun. 2017;8:14234.
Gilman MS, Castellanos CA, Chen M, Ngwuta JO, Goodwin E, Moin SM, et al. Rapid profiling of RSV antibody repertoires from the memory B cells of naturally infected adult donors. Sci Immunol. 2016;1.
Goodwin E, Gilman MSA, Wrapp D, Chen M, Ngwuta JO, Moin SM, et al. Infants Infected with Respiratory Syncytial Virus Generate Potent Neutralizing Antibodies that Lack Somatic Hypermutation. Immunity. 2018;48:339–49 e5.
Doria-Rose NA, Joyce MG. Strategies to guide the antibody affinity maturation process. Curr Opin Virol. 2015;11:137–47.
Eisen HN. Affinity enhancement of antibodies: how low-affinity antibodies produced early in immune responses are followed by high-affinity antibodies later and in memory B-cell responses. Cancer Immunol Res. 2014;2:381–92.
Sliwkowski MX, Mellman I. Antibody therapeutics in cancer. Science. 2013;341:1192–8.
Makabe K, Nakanishi T, Tsumoto K, Tanaka Y, Kondo H, Umetsu M, et al. Thermodynamic consequences of mutations in vernier zone residues of a humanized anti-human epidermal growth factor receptor murine antibody, 528. J Biol Chem. 2008;283:1156–66.
Hoogenboom HR. Selecting and screening recombinant antibody libraries. Nat Biotechnol. 2005;23:1105–16.
Ho M, Kreitman RJ, Onda M, Pastan I. In vitro antibody evolution targeting germline hot spots to increase activity of an anti-CD22 immunotoxin. J Biol Chem. 2005;280:607–17.
Thie H, Voedisch B, Dübel S, Hust M, Schirrmann T. Affinity Maturation by Phage Display. In: Dimitrov AS, editor. Therapeutic Antibodies. Totowa: Humana Press; 2009. p. 309–22.
Steinwand M, Droste P, Frenzel A, Hust M, Dübel S, Schirrmann T. The influence of antibody fragment format on phage display based affinity maturation of IgG. MAbs. 2013;6:204–18.
Article PubMed Central Google Scholar
Low NM, Holliger PH, Winter G. Mimicking somatic hypermutation: affinity maturation of antibodies displayed on bacteriophage using a bacterial mutator strain. J Mol Biol. 1996;260:359–68.
Marks JD. Antibody affinity maturation by chain shuffling. Methods Mol Biol. 2004;248:327–43.
Nielsen UB, Marks JD. Affinity maturation of phage antibodies. In: Clackson T, Lowman HB, editors. Phage Display: A Practical Approach. Oxford: Oxford University Press; 2004. p. 360.
Saunders KO. Conceptual Approaches to Modulating Antibody Effector Functions and Circulation Half-Life. Front Immunol. 2019;10:1296.
Kelley RF, Meng YG. Methods to engineer and identify IgG1 variants with improved FcRn binding or effector function. Methods Mol Biol. 2012;901:277–93.
Liu Z, Gunasekaran K, Wang W, Razinkov V, Sekirov L, Leng E, et al. Asymmetrical Fc engineering greatly enhances antibody-dependent cellular cytotoxicity (ADCC) effector function and stability of the modified antibodies. J Biol Chem. 2014;289:3571–90.
Monnet C, Jorieux S, Souyris N, Zaki O, Jacquet A, Fournier N, et al. Combined glyco- and protein-Fc engineering simultaneously enhance cytotoxicity and half-life of a therapeutic antibody. MAbs. 2014;6:422–36.
Mimura Y, Katoh T, Saldova R, O'Flaherty R, Izumi T, Mimura-Kimura Y, et al. Glycosylation engineering of therapeutic IgG antibodies: challenges for the safety, functionality and efficacy. Protein Cell. 2018;9:47–62.
Li T, DiLillo DJ, Bournazos S, Giddens JP, Ravetch JV, Wang LX. Modulating IgG effector function by Fc glycan engineering. Proc Natl Acad Sci U S A. 2017;114:3485–90.
Chen CL, Hsu JC, Lin CW, Wang CH, Tsai MH, Wu CY, et al. Crystal Structure of a Homogeneous IgG-Fc Glycoform with the N-Glycan Designed to Maximize the Antibody Dependent Cellular Cytotoxicity. ACS Chem Biol. 2017;12:1335–45.
Lin CW, Tsai MH, Li ST, Tsai TI, Chu KC, Liu YC, et al. A common glycan structure on immunoglobulin G for enhancement of effector functions. Proc Natl Acad Sci U S A. 2015;112:10611–6.
Neri D. Antibody-Cytokine Fusions: Versatile Products for the Modulation of Anticancer Immunity. Cancer Immunol Res. 2019;7:348–54.
Beck A, Goetsch L, Dumontet C, Corvaïa N. Strategies and challenges for the next generation of antibody–drug conjugates. Nat Rev Drug Discov. 2017;16:315.
Larson SM, Carrasquillo JA, Cheung N-KV, Press OW. Radioimmunotherapy of human tumours. Nat Rev Cancer. 2015;15:347.
Labrijn AF, Janmaat ML, Reichert JM, Parren PWHI. Bispecific antibodies: a mechanistic review of the pipeline. Nat Rev Drug Discov. 2019.
Ohradanova-Repic A, Nogueira E, Hartl I, Gomes AC, Preto A, Steinhuber E, et al. Fab antibody fragment-functionalized liposomes for specific targeting of antigen-positive cells. Nanomedicine. 2018;14:123–30.
Lu R-M, Chang Y-L, Chen M-S, Wu H-C. Single chain anti-c-Met antibody conjugated nanoparticles for in vivo tumor-targeted imaging and drug delivery. Biomaterials. 2011;32:3265–74.
June CH, Sadelain M. Chimeric Antigen Receptor Therapy. N Engl J Med. 2018;379:64–73.
June CH, O’Connor RS, Kawalekar OU, Ghassemi S, Milone MC. CAR T cell immunotherapy for human cancer. Science. 2018;359:1361–5.
Neelapu SS, Locke FL, Bartlett NL, Lekakis LJ, Miklos DB, Jacobson CA, et al. Axicabtagene Ciloleucel CAR T-Cell Therapy in Refractory Large B-Cell Lymphoma. N Engl J Med. 2017;377:2531–44.
Schuster SJ, Bishop MR, Tam CS, Waller EK, Borchmann P, McGuirk JP, et al. Tisagenlecleucel in Adult Relapsed or Refractory Diffuse Large B-Cell Lymphoma. N Engl J Med. 2019;380:45–56.
Park JH, Rivière I, Gonen M, Wang X, Sénéchal B, Curran KJ, et al. Long-Term Follow-up of CD19 CAR Therapy in Acute Lymphoblastic Leukemia. N Engl J Med. 2018;378:449–59.
Maude SL, Laetsch TW, Buechner J, Rives S, Boyer M, Bittencourt H, et al. Tisagenlecleucel in Children and Young Adults with B-Cell Lymphoblastic Leukemia. N Engl J Med. 2018;378:439–48.
Brudno JN, Kochenderfer JN. Chimeric antigen receptor T-cell therapies for lymphoma. Nat Rev Clin Oncol. 2017;15:31.
Chen IC, Chiu YK, Yu CM, Lee CC, Tung CP, Tsou YL, et al. High throughput discovery of influenza virus neutralizing antibodies from phage-displayed synthetic antibody libraries. Sci Rep. 2017;7:14455.
Barreto K, Maruthachalam BV, Hill W, Hogan D, Sutherland AR, Kusalik A, et al. Next-generation sequencing-guided identification and reconstruction of antibody CDR combinations from phage selection outputs. Nucleic Acids Res. 2019.
Papalexi E, Satija R. Single-cell RNA sequencing to explore immune cell heterogeneity. Nat Rev Immunol. 2017;18:35.
Georgiou G, Ippolito GC, Beausang J, Busse CE, Wardemann H, Quake SR. The promise and challenge of high-throughput sequencing of the antibody repertoire. Nat Biotechnol. 2014;32:158.
Research and Markets. Global and China Monoclonal Antibody Industry Report, 2019–2025. Global, China 2019, April. 180 p.
Download references
Acknowledgements
This work was supported by Academia Sinica and Ministry of Science and Technology [106-0210-01-15-02] and [107-0210-01-19-01], and the Program for Translational Innovation of Biopharmaceutical Development - Technology Supporting Platform Axis and [107-0210-01-19-04], Taiwan (to H.-C. Wu).
Author information
I-Ju Liu, Chi-Chiu Lee and Han-Zen Tsai contributed equally to this work.
Authors and Affiliations
Institute of Cellular and Organismic Biology, Academia Sinica, Taipei, 115, Taiwan
Ruei-Min Lu, Yu-Chyi Hwang, I-Ju Liu, Chi-Chiu Lee, Han-Zen Tsai, Hsin-Jung Li & Han-Chung Wu
128 Academia Rd., Section 2, Nankang, Taipei, 11529, Taiwan
Han-Chung Wu
You can also search for this author in PubMed Google Scholar
Contributions
R-M L, Y-C H, I-J L, C-C L, H-Z T, H-J L, and H-C W wrote the manuscript. R-M L, C-C L, H-Z T, and H-C W designed and illustrated Table. R-M L, Y-C H, I-J L, C-C L, H-Z T, H-J L, and H-C W designed and illustrated figures. All authors read and approved the final manuscript.
Corresponding author
Correspondence to Han-Chung Wu .
Ethics declarations
Ethics approval and consent to participate, consent for publication, competing interests.
The authors declare that they have no competing interests.
Additional information
Publisher’s note.
Springer Nature remains neutral with regard to jurisdictional claims in published maps and institutional affiliations.
Rights and permissions
Open Access This article is distributed under the terms of the Creative Commons Attribution 4.0 International License ( http://creativecommons.org/licenses/by/4.0/ ), which permits unrestricted use, distribution, and reproduction in any medium, provided you give appropriate credit to the original author(s) and the source, provide a link to the Creative Commons license, and indicate if changes were made. The Creative Commons Public Domain Dedication waiver ( http://creativecommons.org/publicdomain/zero/1.0/ ) applies to the data made available in this article, unless otherwise stated.
Reprints and permissions
About this article
Cite this article.
Lu, RM., Hwang, YC., Liu, IJ. et al. Development of therapeutic antibodies for the treatment of diseases. J Biomed Sci 27 , 1 (2020). https://doi.org/10.1186/s12929-019-0592-z
Download citation
Received : 01 August 2019
Accepted : 18 November 2019
Published : 02 January 2020
DOI : https://doi.org/10.1186/s12929-019-0592-z
Share this article
Anyone you share the following link with will be able to read this content:
Sorry, a shareable link is not currently available for this article.
Provided by the Springer Nature SharedIt content-sharing initiative
- Therapeutic antibody
- Antibody market
- Humanized antibody
- Phage display
- Human antibody mouse
- Affinity maturation
Journal of Biomedical Science
ISSN: 1423-0127
- Submission enquiries: Access here and click Contact Us
- General enquiries: [email protected]
An official website of the United States government
Official websites use .gov A .gov website belongs to an official government organization in the United States.
Secure .gov websites use HTTPS A lock ( Lock Locked padlock icon ) or https:// means you've safely connected to the .gov website. Share sensitive information only on official, secure websites.
- Publications
- Account settings
- Advanced Search
- Journal List

Monoclonal Antibodies in Cancer Therapy
David zahavi, louis weiner.
- Author information
- Article notes
- Copyright and License information
Correspondence: [email protected] ; Tel.: +1-202-687-2110
Received 2020 May 22; Accepted 2020 Jul 4; Collection date 2020 Sep.
Licensee MDPI, Basel, Switzerland. This article is an open access article distributed under the terms and conditions of the Creative Commons Attribution (CC BY) license ( http://creativecommons.org/licenses/by/4.0/ ).
Monoclonal antibody-based immunotherapy is now considered to be a main component of cancer therapy, alongside surgery, radiation, and chemotherapy. Monoclonal antibodies possess a diverse set of clinically relevant mechanisms of action. In addition, antibodies can directly target tumor cells while simultaneously promoting the induction of long-lasting anti-tumor immune responses. The multifaceted properties of antibodies as a therapeutic platform have led to the development of new cancer treatment strategies that will have major impacts on cancer care. This review focuses on the known mechanisms of action, current clinical applications for the treatment of cancer, and mechanisms of resistance of monoclonal antibody therapy. We further discuss how monoclonal antibody-based strategies have moved towards enhancing anti-tumor immune responses by targeting immune cells instead of tumor antigens as well as some of the current combination therapies.
Keywords: monoclonal antibody, cancer, immunology, antibody-dependent cellular cytotoxicity (ADCC), immune checkpoint blockade (ICB)
1. Introduction
Antibodies were first described as a neutralizing substance found in blood by Behring and Shibasaburo in 1890 in their work on animal models of diphtheria [ 1 ]. Over the next century several important scientific advances would pave the way for the use of antibodies as a cancer therapeutic. Antibodies were identified as proteins that could recognize specific antigens by Heidelberger and Avery, and in 1947 Astrid Fagraeus demonstrated that antibodies were produced by plasma B cells of the adaptive immune system [ 2 , 3 ]. Sir Gustav Nossal then proved the clonal selection theory that a single B cell clone produces one specific antibody [ 4 ]. Thus, monoclonal antibodies (mAbs) are antibodies made by clones of a unique B cell, all of which bind to specific portions of an antigen also known as an epitope. Methods to produce monoclonal antibodies involving human–mouse hybrid cells were first identified by Schwaber in 1973 and were used by Köhler and Milstein to generate the human-derived hybridomas that have since been a mainstay in the large scale production of therapeutic antibodies [ 5 , 6 ]. Soon after the discovery of hybridomas, research into the use of mAbs to treat cancer began. Anti-melanoma mAbs were shown to suppress the growth of human melanomas in nude mice and in 1980 the first human trial of mAb therapy against cancer was conducted in a lymphoma patient [ 7 , 8 ]. Unfortunately, due to the murine origins of early therapeutic monoclonal antibodies, these mAbs were immunogenic in humans and were poor inducers of immunity in patients, thereby limiting their clinical applicability. In the late 1980s, techniques emerged to humanize antibodies in order to eliminate these limitations [ 9 ]. Further advances have led to the derivation of “fully-human” antibodies using transgenic mice or in vitro yeast or phage display systems [ 10 , 11 ]. As a result of these antibody engineering innovations, mAbs have become an important modality in the treatment of cancer. Antibodies are unique in their ability to both directly kill tumor cells while simultaneously engage the host immune system to develop long-lasting effector responses against the tumor. The combination of a multifaceted mechanism of action with target specificity distinguishes mAb therapy from treatments such as chemotherapy and underlies the capability of antibodies to elicit strong anti-tumor responses while minimizing toxicity and adverse events. To better understand how monoclonal antibodies function as a cancer therapy, we review their structure, mechanisms of action, clinical uses, mechanisms of resistance, and some combination strategies.
2. Antibody Structure and Function
Antibodies are large glycoproteins that belong to the immunoglobulin (Ig) superfamily and their role in the immune system is to recognize foreign antigens, neutralize them, and elicit a further immune response. Their basic structure is composed of two heavy and two light chains in the shape of a Y. At each tip of the Y lies the fragment antigen-binding (Fab) portion of the antibody which is responsible for recognition of the specific antigen. The fragment crystallizable (Fc) region located at the base of the Y structure mediates interactions between the antibody and other members of the immune system [ 12 ]. Antibody Fc regions are recognized by Fc receptors (FcRs) found on a wide range of immune cells. Based on the type of heavy chain, antibodies can be separated into five distinct classes: IgA, IgD, IgE, IgG, and IgM. IgG is the most often form used in antibody therapy due to the fact that IgGs interact with their associated type of FcR, FcγR, found on natural killer (NK) cells as well as neutrophils, monocytes, dendritic cells, and eosinophils to mediate specialized functions such as antibody-dependent cellular cytotoxicity (ADCC) and complement-dependent cytotoxicity (CDC). The IgG class can further be subdivided based on the ability of the Fc region to facilitate those functions: IgG1 and IgG3 are able to elicit ADCC and CDC, while IgG2 and IgG4 cannot [ 13 ]. Monoclonal antibodies represent a clonal version of a specified antibody isotype that is targeted to a unique antigen epitope.
3. Effector Mechanisms of Targeted mAbs
Targeted mAbs against antigens either unique to or overexpressed by tumor cells can cause tumor cell death by a variety mechanisms ( Figure 1 ). The main direct mechanism by which many antibodies induce tumor cell death is the blockade of growth factor receptor signaling. Pro-tumor growth and survival signaling is perturbed when mAbs bind their target growth factor receptors and manipulate their activation state or block ligand binding. For example, epidermal growth factor receptor (EGFR) is overexpressed by many different cancers and signaling via EGFR leads to tumor cell proliferation, migration, and invasion. Cetuximab, which is an anti-EGFR mAb, induces apoptosis in tumor cells by blocking ligand binding and receptor dimerization [ 14 , 15 ]. Human epidermal growth factor receptor 2 (HER2) is a tyrosine kinase receptor that is overexpressed in many cancers but primarily ovarian and breast carcinomas [ 16 ]. It is distinct from EGFR in that it has no known ligand and instead hetero-dimerizes with other growth factor receptors to enhance their activation [ 17 ]. Antibodies targeting HER2 therefore achieve signaling perturbation by inhibiting hetero-dimerization and internalization. Trastuzumab was the first FDA approved anti-HER2 mAb and remains a vital component of treatments for HER2 -amplified breast cancer. Indirect mechanisms of action of mAbs require the engagement of components of the host immune system and are CDC, antibody-dependent cellular phagocytosis (ADCP), and ADCC. Most targeted mAbs are able to activate the complement system. For instance, rituximab depends in part on CDC for its in vivo efficacy. In a preclinical model, rituximab anti-tumor effects were completely abolished by knockout of the complement cascade component C1q [ 18 ]. The importance of CDC in mAb therapy is further supported by the fact that genetic polymorphisms in the C1qA gene correlate with clinical response to rituximab in patients with follicular lymphoma [ 19 ]. Likewise, optimization of CDC via antibody engineering can enhance anti-tumor activity. For example, the anti-CD20 mAb ofatumumab, which mediates amplified CDC, demonstrated greater efficacy than rituximab in a clinical trial of chronic lymphocytic leukemia (CLL) patients [ 20 ]. ADCP occurs when FcγRI expressed on cells such as macrophages binds to IgG1 or IgG3 mAbs that have opsonized a tumor cell. There have been very limited studies of ADCP; however, there is some evidence that ADCP plays an important role in destruction of circulating tumor cells following mAb therapy [ 21 ]. First described in 1965 by Erna Möeller, ADCC has since been established as an immune mechanism where target cells become opsonized by antibodies which then recruits effector cells to induce target cell death by non-phagocytic mechanisms [ 22 ]. Antibodies act as bridges between by binding to antigens on the target cell surface via their Fab portions and linking the effector cells via their Fc portions. While IgG, IgA, and IgE can all mediate ADCC, IgG1 is the most relevant subclass for anti-cancer therapeutic antibodies [ 23 ]. Effector cells must express FcR that will bind the antibody in order to facilitate ADCC [ 24 ]. Each class of antibody has a corresponding class of FcR such as FcγR, which binds IgG, and FcαR, which binds IgA. FcγR is the most relevant class to ADCC of tumor cells and encompasses both the activating FcγRI (CD64), FcγRIIA (CD32A), FcγRIIIA (CD16A), and inhibitory FcγRIIB (CD32B) receptors [ 25 ]. When an activating FcγR on an effector cell binds the Fc region of an antibody receptor crosslinking and downstream signal propagation occurs. NK cells are the main effector type that mediate ADCC; however other myeloid types such as monocytes, macrophages, neutrophils, eosinophils, and dendritic cells are also capable [ 26 ]. Effector cells induce target cell death via cytotoxic granule release, Fas signaling, and initiation of reactive oxygen species [ 27 , 28 , 29 ]. While several myeloid cell types have been demonstrated to mediate ADCC during immunotherapy, the clinical efficacy of most targeted mAbs is mainly NK cell dependent [ 30 ].
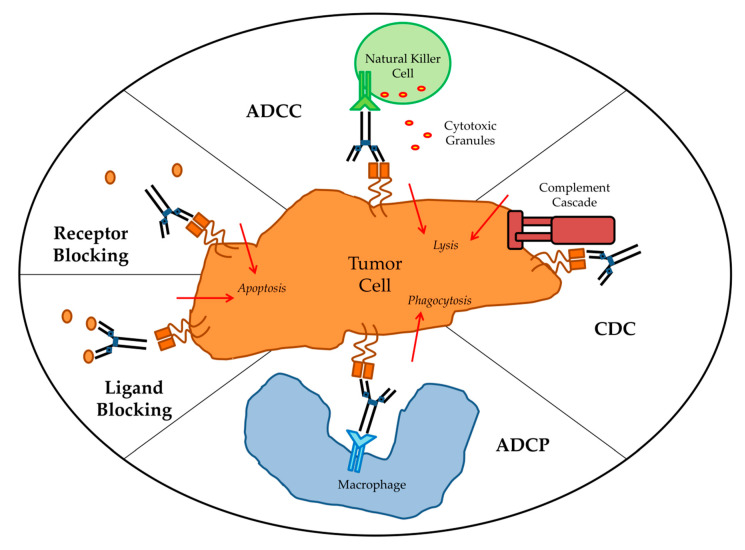
Antibody effector mechanisms. ADCC: antibody-dependent cellular cytotoxicity; CDC: complement-dependent cytotoxicity; ADCP: antibody-dependent cellular phagocytosis.
While many mAbs facilitate several of the above mechanisms there has been debate about which mechanisms are important in vivo. Many of the first mAb therapies were known to mediate ADCC of tumor cells in vitro, but whether ADCC was significant to their therapeutic efficacy was originally poorly understood. Using mouse models, Clynes et al. were the first to demonstrate that ADCC was an essential contributor to the in vivo activity of trastuzumab and rituximab [ 31 ]. Additional mechanistic studies utilizing similar mouse models confirmed that FcγR expression by immune effector cells is required for tumors to respond to mAb therapy [ 32 ]. Furthermore, in a novel mouse model whose immune cells had mutant FcγR incapable of ADCC, mAb therapy failed to clear tumors [ 33 ]. In most of these studies the mAbs used could also act through additional mechanisms of action such as signaling perturbation. Therefore, while these results indicated ADCC was required for successful mAb therapy they did not prove whether ADCC alone was sufficient. More recent work involving mAbs that rely exclusively on ADCC have verified that ADCC alone can mediate therapeutic benefit [ 34 ]. In humans, clinical trials have demonstrated that many mAbs eliminate tumor cells, in part, by causing ADCC. Because functioning FcγRs were critical for mAb efficacy in mouse models, clinical trial data was used to examine whether FcγR polymorphisms would correlate with clinical outcomes. In humans, bothFcγRIIA and FcγRIIIA are polymorphic, with certain genotypes coding for FcγRs with higher affinity for IgG1 and therefore stronger ADCC activity [ 35 , 36 ]. Lymphoma patients who had the polymorphism associated with augmented ADCC were demonstrated to have better clinical responses to rituximab in several studies [ 37 , 38 , 39 ]. Furthermore, both genotypes of FcγRIIA and FcγRIIIA associated with higher affinity for Fc were robust predictors for better survival in colorectal cancer patients treated with cetuximab and metastatic breast cancer patients treated with trastuzumab respectively [ 40 , 41 ]. In recent studies, FcγR polymorphisms in breast cancer patients treated with trastuzumab or neuroblastoma patients treated with anti-GD2 mAbs were directly linked to ADCC amplitude by in vitro studies using patient derived immune cells [ 42 , 43 ]. These multiple analyses confirm that patients with high affinity FcγR that mediate stronger ADCC have better clinical outcomes when administered mAb therapy, irrespective of cancer type or target antigen. In addition to examining FcγR polymorphisms, studies have used patient samples from clinical trials to investigate the relative importance of ADCC to therapeutic success. In one study, patients with HER2-positive breast cancer that were treated with trastuzumab had IHC staining for granzyme B performed on their tumor samples as a surrogate marker of ADCC activity. Patients who received trastuzumab were found to have better overall survival and higher levels of ADCC compared to the other cohorts [ 44 ]. Furthermore, patient-derived in vitro models demonstrated ADCC as a major therapeutic mechanism of rituximab in non-Hodgkin lymphoma and anti-CD38 antibodies in multiple myeloma [ 45 , 46 ]. Taken together, there is strong evidence that ADCC plays a critical role in facilitating mAb-based anti-tumor therapeutic responses in patients. In fact, additional variables that would affect ADCC activity such as target antigen expression level and density, mAb isotype, and mAb dose all correlate with clinical response [ 47 ]. The ability of mAbs to mediate ADCC is recognized as a major determining factor for mAb therapy success, and research and development of novel mAbs has shifted towards designing mAbs with improved capacity to mediate ADCC. ADCC functionality of antibodies can be enhanced by altering the Fc portion of the mAb to increase their binding affinity to the activating FcγRIIIA via site-directed mutagenesis, changing Fc domain glycosylation, and/or removing Fc domain fucosylation [ 48 , 49 , 50 , 51 ]. Next generation mAbs that are afucosylated have shown promise in clinical trials [ 52 ].
4. Clinical Uses
Over the past thirty years, various forms of mAb-derived treatments have been used clinically in an effort to capitalize on the potential of targeted therapy. Antibodies are extremely versatile as platforms for the development of novel therapeutics which has resulted in a large diversity of approaches. The discovery of targetable tumor-specific antigens fueled interest in designing immunotherapies [ 53 ]. Upon the emergence of mAbs, it was thought that using mAbs to target tumor cell antigens might be a more effective and less toxic treatment than traditional chemotherapy. In 1988, scientists identified a protein, CD20, which was specific to mature B cells. CD20 was found to be abundantly expressed on cancerous B cells in non-Hodgkin’s lymphoma, but not found on healthy immature B cells. Therefore, a mAb treatment that targeted CD20 could eliminate the cancerous cells, but immature B cells would remain to replenish the supply of healthy cells. Thus, CD20 became the first target for mAb therapy and the anti-CD20 mAb rituximab was the first mAb to be approved for the treatment of cancer [ 54 ]. By targeting antigens identified as overexpressed on solid tumor cells, more mAbs have since demonstrated efficacy as cancer therapeutics. Today, mAbs directed to such targets as epidermal growth EGFR and HER2 see wide use in the clinic for the treatment of colorectal and breast cancers respectively [ 55 , 56 ]. In addition, there is currently a multitude of other ways that mAbs are employed in cancer therapy including antibody-drug conjugates, targeting pro-tumorigenic compounds in the microenvironment, bispecific T cell engagers (BiTEs), and immune checkpoint inhibitors. A comprehensive list of FDA approved mAb-based cancer therapies is provided in Table 1 .
FDA-approved monoclonal antibodies for cancer.
1 Indications and year of first approval for each antibody were accessed using the FDA drug database [ 57 ].
Early efforts in the development of mAb therapies focused on enhancing the direct cytotoxic effects on targeted tumor cells. With the exception of the aforementioned mAbs directed against CD20, HER2, and EGFR, most mAbs have little anti-tumor activity on their own. In spite of this, the specificity of mAbs for tumor antigen makes them useful for delivering cytotoxic compounds directly to tumor cells. Clinically beneficial anti-tumor activity has been accomplished by conjugating mAbs with different effector molecules that cause tumor cell death after antibody binding and internalization. Effector molecules may include cytotoxic drugs, immunotoxins, and radionuclide agents. The most important consideration in antibody–conjugate design is the selection of the target, which is the main determinant of anti-tumor activity and selectivity [ 58 ]. In addition, targets must be capable of internalization upon antibody binding in order to release the drug. Brentuximab vedotin became the first antibody–drug conjugate (ADC) to be FDA approved in 2011 [ 59 ]. Brentuximab vedotin is a mAb targeting CD30 expressed by lymphoma cells linked to the microtubule destabilizing agent monomethyl auristatin E. Ado-trastuzumab emtansine is an ADC composed of trastuzumab and the cytotoxic maytansine derivative DM1 that was approved in 2013 for patients with metastatic breast cancer that is HER2-positive [ 60 ]. Eight ADCs have been approved for use in the treatment of various cancers ( Table 1 ). ADCs targeting mesothelin, DLL3, and GPNMB, among others remain in advanced stages of clinical testing [ 61 ]. Research into ADCs continues because of improved understanding of the mechanistic basis of ADC activity that enables rational design of combinations with other cytotoxic payloads and findings indicate that ADCs may also stimulate additional anti-tumor immunity by T cells [ 62 ]. The second class of agents that can be delivered to tumor cells via conjugation to mAbs are biologic toxins. This method has proven difficult due to the extreme potency of the toxins, causing unacceptable toxicity in patients. Pseudomonas exotoxin A (PE) and ricin toxins are the most common toxins in targeted cancer therapy and remain under clinical investigation [ 63 ]. To date, only moxetumomab pasudotox, a CD22 targeted mAb linked to PE for hairy-cell leukemia, has received FDA approval [ 64 ]. The last category of antibody-based compound delivery involves radionuclides. Radioimmunotherapy uses a mAb labeled with a radionuclide as a form of targeted radiation therapy. Currently, only two radioimmunotherapies have been FDA approved: yttrium-90 (90Y)-ibritumomab tiuxetan and iodine-131 (131I)–tositumomab. Both agents utilize a mAb specific for CD20 to deliver either yttrium-90 or iodine-131 to lymphoma cells. Unfortunately, radioimmunotherapies can cause life-threatening systemic toxicity and solid tumors are often inaccessible or insensitive. Since the practicalities of preparing and delivering these agents have proved complex they have not seen widespread use, and tositumomab was discontinued by their parent company [ 65 ].
The tumor microenvironment contains many factors that are known to inhibit anti-tumor immune responses, promote tumor cell growth, and induce pro-tumorigenic angiogenesis. Targeting these critical pro-tumorigenic processes within the tumor microenvironment has proved clinically efficacious. Historically, the most relevant target has been vascular endothelial growth factor (VEGF), which is abundant in the microenvironment of many solid tumors and binds to its receptor (VEGFR) found on the tumor adjacent vascular endothelium to stimulate angiogenesis. The mAb bevacizumab, which is targets VEGF and blocks VEGF from binding to its receptor, is approved for the treatment of many different cancers [ 66 ]. Similar efforts to target VEGFRs using ramucirumab, a mAb to VEGFR2, and icrucumab, a mAb to VEGFR1, have shown promise [ 67 , 68 ]. Other pathways and factors that are proangiogenic such as platelet-derived growth factor (PDGF)/PDGF-receptor (PDGFR) signaling are important therapeutic targets [ 69 ]. In addition to proangiogenic targets, transforming growth factor-beta (TGF-β), which is secreted by some tumor cells, inhibits immune effector cell function in the tumor microenvironment [ 70 ]. Fresolimumab is a mAb that targets TGF-β and is in ongoing clinical trials [ 71 ]. Targeting the tumor microenvironment with mAbs represents a compelling strategy to synergistically inhibit pro-tumorigenic processes when combined with tumor targeted therapy.
Recently, the most successful mAb-based strategies have moved away from targeting tumor antigens and instead focused on targeting immune cells in order to enhance their anti-tumor capabilities. One of the first mAb approaches to stimulate T cell anti-tumor immunity was the development of bispecific T Cell Engager (BiTE) antibodies that both target a tumor antigen such as CD19 and the activating receptor, CD3, on T cells. BiTEs combine direct targeting of tumor cells with recruitment of cytotoxic T cells into the tumor microenvironment and led to tumor regressions even when administered at doses three orders of magnitude less than the parent mAb alone [ 72 ]. The CD19-CD3 BiTE blinatumomab conferred significant clinical benefit to acute lymphoblastic leukemia patients and was FDA approved in 2017 [ 73 ]. Clinical trials are currently underway using BiTEs generated from the widely used anti-HER2 and anti-EGFR mAbs trastuzumab and cetuximab. Other mAb approaches seek to enhance T cell specific immunity against tumor cells by stimulating activating receptors such as 4-1BB, OX40, CD27, CD40, and ICOS ( Figure 2 ). Agonist antibodies towards CD40 stimulate antigen presentation by dendritic cells and mAbs to OX40 and 4-1BB activate T cells while simultaneously dampening the activity of inhibitory T regulatory cells (Tregs) [ 74 ]. mAbs designed to stimulate these activating receptors are in various stages of clinical trials both alone and in combination with other immunotherapy approaches. Additional mAbs that deplete inhibitory Tregs directly, such as daclizumab, which targets CD25 on Tregs, are also undergoing clinical trials [ 75 ].
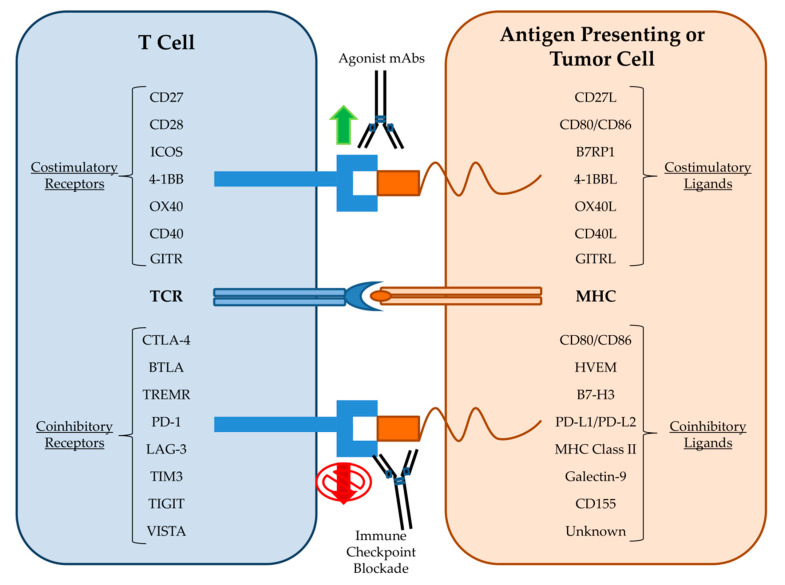
Immune checkpoint targets of monoclonal antibodies.
The most well-known and promising type of mAb therapy for cancer is the blockade of immune checkpoints ( Figure 2 ). Immune cell activation and regulation is a highly complex process that must integrate a variety of costimulatory and coinhibitory signals in order to control immune cell responses to antigen. Immune checkpoints are inhibitory receptors and pathways that are responsible for maintaining self-tolerance and modulating immune responses in order to curtail collateral tissue damage [ 76 ]. Cytotoxic T lymphocyte antigen-4 (CTLA-4) was the first T cell checkpoint to be identified. CTLA-4 is mainly expressed by Treg cells but also becomes upregulated on activated T cells where it then outcompetes for binding of the costimulatory ligands CD80 and CD86 [ 77 ]. Therefore, it was theorized that blockade of CTLA-4 could indirectly and directly amplify the anti-tumor T cell response by removing inhibitory Tregs and maintaining the activating signals to cytotoxic T cells respectively. Immune checkpoint blockade (ICB) therapy utilizing mAbs against CTLA-4 was then introduced and following success in animal models was rapidly developed for evaluation in clinical trials [ 78 ]. In 2011, the FDA approved the first ICB therapy, the anti-CTLA-4 mAb Ipilimumab, based on promising results from a clinical trial in melanoma patients [ 79 ]. Ipilimumab remains the subject of clinical trials for use in additional cancer types [ 80 ]. Programmed death receptor-1 (PD-1) is another inhibitory immune checkpoint and is associated with the programmed death pathway in T cells [ 81 ]. PD-1 is expressed on activated CD8+ T cells, Tregs, and activated B cell and natural killer (NK) cells. PD-1 is considered a major regulator of effector T cell function and is therefore regarded as a key checkpoint target [ 82 ]. Tumor cells are known to upregulate the PD-1 ligand, PD-L1, in order to exhaust tumor infiltrating lymphocytes (TILs) [ 83 ]. In 2014, the anti-PD-1 mAb nivolumab gained FDA approval for melanoma patients following reports of improved patient outcomes in the CheckMate-037 clinical trial [ 84 ]. Subsequent clinical trial successes led to the approval of nivolumab and another anti-PD-1 mAb, pembrolizumab, for the treatment of a wide variety of malignancies [ 85 , 86 , 87 ]. Additional mAbs targeting either PD-1 (pidilizumab) or its ligands (durvalumab and atezolizumab) have also performed well in clinical trials [ 88 ]. As of this writing, mAbs against the immune checkpoints CTLA-4, PD-1, and PD-L1 have received numerous FDA approvals and are used as first-line therapies for the treatment of certain solid tumors [ 89 ]. The robust effectiveness of ICB has led to the rapid study of other immune cell inhibitory receptors. Members of the immunoglobulin superfamily such as lymphocyte activation gene 3 (LAG3), T cell immunoglobulin and mucin domain-containing 3 (TIM3), T cell immunoglobulin and immunoreceptor tyrosine-based inhibitory motif domain (TIGIT), and V-domain Ig suppressor of T cell activation (VISTA) are all being explored as potential checkpoint therapeutic targets [ 90 , 91 ]. Importantly, checkpoint blockade also affects other components of the innate immune system such as NK cells. NK cells have the intrinsic ability to kill tumor cells; however, NK cell effector function is modulated by various molecular checkpoints [ 92 ]. The blockade of NK cell associated inhibitory receptors such as KIRs are therefore under preclinical investigation [ 93 ]. The demonstrable anti-tumor activity and favorable toxicity profile of ICB has cemented mAbs as one of the backbones of cancer therapy.
5. Mechanisms of Resistance
Although mAb therapy has proven successful in the treatment of cancer, clinical resistance to these agents continues to be a major issue. Only a minority of patients will respond, with the vast majority developing refractory disease within one year [ 94 , 95 , 96 ]. Therapeutic resistance can be considered either innate (primary) or acquired (secondary) with differing mechanisms in each scenario. Innate resistance is mainly due to mutations already present in the tumor cells prior to therapy whereas acquired resistance is the result of immune selection pressure and immunoediting of the tumor during therapy. Preclinical models and clinical trials of mAb therapy have unraveled a myriad of mechanisms of resistance; and they include: Mutations of the antibody target, induction of alternative growth signaling pathways, epithelial to mesenchymal transition (EMT), and impaired effector cell responses.
A limitation of mAb therapy is that efficacy is dependent on tumor cell expression of the target molecules that are able to be bound by the antibodies. While CD20 gene mutations can confer irreversible resistance to rituximab in lymphoma patients, such mutations were rarely detected at both the initiation of treatment and in cases who relapsed following therapy [ 97 ]. A S492R mutation in the EGFR ectodomain imparts resistance to cetuximab but not panitumumab due to their recognition of distinct epitopes [ 98 ]. Interestingly, cancer cells expressing EGFR variant III are less sensitive to cetuximab even though the cetuximab binding epitope remains intact [ 99 ]. Cell lines chronically exposed to rituximab acquire resistance that is associated with the downregulation of CD20 at both the transcriptional and protein level [ 100 ]. Likewise, multiple myeloma patients who received the anti-CD38 monoclonal antibody daratumumab lost CD38 expression in their tumors which correlated with impaired response [ 101 ]. Cetuximab-mediated ADCC highly correlates with EGFR surface expression in cell lines but clinical response in patients appear to be independent of tumor EGFR expression level [ 102 , 103 ]. Instead, it has been suggested that mutations and polymorphisms of EGFR are responsible for cetuximab refractory disease. In HNSCC patients that expressed the EGFR-K521 variant (~40% of cases) there was reduced affinity of cetuximab to EGFR and efficacy could only be restored with optimization of ADCC [ 104 ]. Similarly, KRAS mutation status may affect susceptibility of EGFR overexpressing cancers to ADCC. Cell lines with mutant KRAS had impaired Fas–Fas ligand interactions that are necessary for induction of target cell apoptosis during ADCC [ 105 ]. Downregulation of HER2 expression has been proposed as a mechanism of resistance to trastuzumab-mediated ADCC but it remains a controversial issue [ 106 ]. Despite conflicting results from in vitro studies there was no reduction found in HER2 expression in breast cancer patients who received trastuzumab [ 107 ]. However, it is known that interferon gamma (IFNγ) exposure can lead to HER2 downregulation through STAT1 mediated pathways [ 108 ]. Furthermore, trastuzumab-mediated ADCC induces IFNγ release from NK cells which leads to a STAT1 dependent downregulation of HER2 expression and concomitant resistance to trastuzumab [ 109 ]. It is also known that IFNγ-induced activation of STAT1 signaling leads to PD-L1 upregulation on the tumor cell surface that confers resistance to NK cell-mediated ADCC [ 110 ].
Mutations of the antibody target and associated downstream signaling molecules can lead to acquired resistance to mAb therapy by activating alternative growth or survival signaling pathways. In colorectal cancers, the most frequent mechanism of cetuximab resistance has been reported as genomic alterations in downstream effectors of EGFR such as KRAS , NRAS , BRAF , and PIK3CA [ 111 ]. Alterations in these pathways bypass EGFR signaling inhibition by cetuximab. For example, KRAS point mutations are causally linked with acquired resistance to cetuximab treatment in colorectal cancer [ 112 ]. In metastatic colorectal cancer patients, activating mutations of the oncogenes RAS , BRAF , and/or PIK3CA were identified as significant predictors of primary resistance to cetuximab [ 113 ]. The resulting enhanced signaling through the downstream MAPK and PI3K/AKT pathways and increased expression of anti-apoptotic BCL-2 proteins is a main mechanism of resistance to mAb induced apoptosis. Furthermore, NRAS mutations that maintain MAPK signaling prevent cetuximab efficacy by preserving the dysregulated ligandless signaling of the pro-tumorigenic EphA2 receptor [ 114 ]. Activation of alternative proliferative and survival pathways such as MAPK and eIF5A2 has also been discovered in HNSCC and hepatocellular carcinoma respectively in response to cetuximab [ 115 , 116 ]. HER2 mutations in breast cancer can confer resistance to trastuzumab; however, trastuzumab is still able to bind the mutant HER2 [ 117 ]. Mutant HER2 leads to dysregulation of the PI3K-AKT signaling pathway and enables trastuzumab resistance through similar anti-apoptotic effector molecules. Furthermore, activating mutations of the PI3K/AKT/mTOR pathway also contribute to trastuzumab resistance in breast cancer [ 118 ]. Several studies have reported overexpression of compensatory growth factors such as insulin-like growth factor-I receptor or EGFR as additional potential mechanisms of resistance to trastuzumab [ 119 ]. Other signaling pathways implicated in trastuzumab resistance include aberrant activation of the tyrosine kinase SRC, cyclin E/cyclin-dependent kinase (CDK) 2, and cyclin D1/CDK4/6 [ 120 ]. In one study of esophageal squamous cell carcinoma, trastuzumab resistant tumor clones had a reduced susceptibility to the perforin-granzyme system [ 121 ]. Similarly, X-linked inhibitor of apoptosis protein, which is overexpressed in breast cancer, drove resistance to ADCC mediated by both cetuximab and trastuzumab [ 122 ]. In an in vitro study of rituximab-resistant lymphoma clones, major survival pathways such as NF-κB and ERK1/2 became constitutively hyper-activated after treatment, which led to overexpression of factors such as Bcl-2, Bcl-xL, and Mcl-1 that prevented the induction of apoptosis by rituximab [ 123 ].
Epithelial to mesenchymal transition (EMT) is a process in which cancer cells lose their epithelial phenotype characterized by cell-to-cell adhesion and instead gain the invasive properties of mesenchymal cells. In preclinical models, EMT was uncovered as a possible mechanism of cetuximab resistance [ 124 ]. The induction of EMT was later confirmed to occur early on in head and neck cancer patients receiving cetuximab. Multiple subsequent studies have revealed EMT mediates acquired resistance to cetuximab via a myriad of mechanisms which include loss of EGFR expression [ 125 , 126 , 127 ]. Moreover, activation of the EMT pathway is a key predictor of cetuximab resistance in colorectal cancer [ 128 ]. In breast cancer, molecular features associated with EMT are linked to primary resistance to trastuzumab [ 129 ]. Additionally, sustained treatment of HER2 positive/PTEN negative breast cancers with trastuzumab induced EMT in a subset of patients which conferred acquired resistance [ 130 ].
ADCC is considered a main therapeutic mechanism of mAb therapy, and clinical resistance often involves impaired cytotoxic immune effector cell responses. Capuano et al. described a novel mechanism of immune exhaustion, whereby NK cells chronically exposed to rituximab lost their cytotoxic functions due to CD16 ligation [ 131 ]. NK cell checkpoints can also regulate ADCC. Poliovirus receptor-like receptors such as TIGIT are known to be involved in trastuzumab-mediated ADCC of cancer cells by NK cells, and blockade of those receptors was able to enhance trastuzumab based responses in breast cancer patients [ 132 ]. Finally, NK cell-mediated ADCC is also dependent on the expression of several proteins that are important members of the immune synapse. ADCC is partly dependent on recognition through ICAM-1 and CD18, though this appears to be less important for trastuzumab-mediated ADCC [ 133 ]. Cancer cell loss of ligands for the activating NKG2D receptor on NK cells such as MICA and dysregulation of the NKG2A-HLA-E axis can also prevent NK cell initiation of ADCC [ 134 ]. A recently reported novel mechanism of resistance to ADCC involves the downregulation of multiple cell surface proteins associated with the immune synapse in response to cetuximab and trastuzumab [ 135 ].
6. Combination Therapies
While mAb has success as a monotherapy in some patients, treatment paradigms are trending towards employing them as combinations with chemotherapy, radiation, molecularly targeted drugs such as tyrosine kinase inhibitors, other antibodies against the same target, immune checkpoint inhibitors, vaccines, and/or cellular therapies. These many combination strategies are currently undergoing both preclinical investigation and clinical trials and this vast field is more exhaustively covered elsewhere [ 136 ]. In this section, we will briefly cover combination therapies involving multiple monoclonal antibodies. It is now widely recognized that the mechanism of action of monoclonal antibodies includes an immune effector cell component. In particular, cetuximab efficacy has been partly attributed to ADCC, which can link innate and adaptive anti-tumor immune responses. Destruction of tumor cells via NK cell-mediated ADCC releases tumor cell specific proteins that when presented by antigen presenting cells to cytotoxic T cells leads to a more effective anti-tumor response. Head and neck squamous cell carcinoma (HNSCC) patients with durable responses to cetuximab have sustained anti-tumor specific immune responses [ 137 ]. With the rise of immune checkpoint inhibitors that can further potentiate such immune responses, it is hypothesized that ICB may act in a synergistic manner with cetuximab. There is growing to support combining anti-PD-1/PD-L1 mAbs with cetuximab in HNSCC patients [ 138 ]. Additionally, combinations of either pembrolizumab or avelumab with cetuximab are currently in clinical trials [ NCT03082534 , NCT03082534 ]. Likewise, the use of ICB in breast cancer in order to enhance anti-HER2 mAb therapies is a promising strategy. In fact, preclinical evidence suggests that resistance to trastuzumab monotherapy can be overcome by combination with ICB [ 139 ]. Based on those results several clinical trials were formed to investigate the relationship between ICB and HER2-targeted mAbs [ 140 ]. Preliminary results from the phase I/II PANACEA trial, which tested pembrolizumab combined with trastuzumab in treating breast cancer patients who overexpressed HER2, indicated synergy in the PD-L1+ patient subset [ 141 ].
Although there are many immune checkpoints of T-cell activation, each checkpoint has distinct mechanisms. Consequently, ICB combinations that target multiple checkpoints will enhance T cell responses in a synergistic manner. The combination of mAbs targeting CTLA-4 and PD-1 performed significantly better in preclinical mouse models than either antibody alone [ 142 ]. Similarly, in metastatic melanoma patients combined therapy of ipilimumab and nivolumab was found to be more effective than either treatment used as a monotherapy [ 143 ]. The FDA has since approved the combination of ipilimumab and nivolumab for melanoma. As the first ICB combination with FDA approval, ongoing clinical trials continue to evaluate ipilimumab plus nivolumab in other cancer types.
Anti-PD-1 mAbs are most often used in combinatorial strategies due to their more favorable toxicity profile in contrast to anti-CTLA-4 monoclonal antibodies. The immune checkpoints LAG3 and TIM3 are commonly found co-expressed with PD-1 on exhausted T cells. ICB of LAG3 combined with anti-PD-1 is undergoing clinical trial in glioblastoma ( NCT02658981 ) and other cancers ( NCT02460224 ). There are similar clinical trials for the combination of anti-TIM3 and anti-PD-1 antibodies in liver cancer ( NCT03680508 ) and several other solid tumors ( NCT03744468 ). Another promising combination strategy involves uniting ICB with agonistic antibodies that activate stimulatory receptors. 4-1BB is a costimulatory receptor found on T cells and NK cells and clinical trials that evaluate 4-1BB agonist antibodies in combination with anti-PD-1 mAb therapy are underway ( NCT02253992 and NCT02179918 ). An agonist antibody to the glucocorticoid-induced tumor necrosis factor receptor–related protein (GITR), which promotes T cell activation, also proved to be successful when combined with nivolumab [ 144 ]. Additional mAb combinations that include agonist antibodies to OX40, which only becomes expressed on activated T cells, are the subject of multiple clinical trials ( NCT01714739 and NCT01750580 ).
7. Concluding Remarks
Monoclonal antibody therapy has only recently become one of the major modalities for the treatment of cancer. Many of the mechanisms of action and their clinical relevance remain poorly understood. Despite the notable clinical successes of antibody therapy, therapeutic resistance remains a major challenge. Future studies must focus on analysis of the mechanisms of action of mAbs in order to identify new approaches to increase clinical efficacy. For instance, studies have revealed that ADCC plays a major role in mediating mAb responses and therefore engineering strategies that augment ADCC activity represents a promising future approach. Combinations of tumor targeted mAbs with ICB have demonstrated that there are several encouraging avenues for maximizing the clinical benefit of mAb therapy. Additionally, mutations of both the antibody target and any associated signaling pathways are important biomarkers of mAb efficacy and resistance. Future mAb treatment strategies must incorporate inhibitors of these alternative signaling pathways in order to abrogate resistance. Treatment paradigms involving monoclonal antibodies will continue to evolve and have the potential to offer curative therapy for many cancer patients.
Author Contributions
Conceptualization, D.Z. and L.W.; writing—original draft preparation, D.Z.; writing—review and editing, L.W..; funding acquisition, L.W. Both authors have read and agreed to the published version of the manuscript.
This research was funded by National Institutes of Health, grant number CA50633 and CA51008 (both to LMW).
Conflicts of Interest
The authors declare no conflict of interest.
- 1. Behring E.V. Ueber das Zustandekommen der Diphtherie-Immunitüt und der Tetanus-Immunitüt bei Thieren. Dtsch. Med. Wochenschr. 1890 doi: 10.1055/s-0029-1207589. [ DOI ] [ PubMed ] [ Google Scholar ]
- 2. Van Epps H.L. How Heidelberger and Avery Sweetened Immunology. J. Exp. Med. 2005;202:1306. doi: 10.1084/jem20210fta. [ DOI ] [ PMC free article ] [ PubMed ] [ Google Scholar ]
- 3. Fagraeus A. Plasma Cellular Reaction and Its Relation to the Formation of Antibodies in Vitro. Nature. 1947;159:499. doi: 10.1038/159499a0. [ DOI ] [ PubMed ] [ Google Scholar ]
- 4. Nossal G.J.V., Lederberg J. Antibody Production by Single Cells. Nature. :1958. doi: 10.1038/1811419a0. [ DOI ] [ PubMed ] [ Google Scholar ]
- 5. Schwaber J., Cohen E.P. Human × Mouse Somatic Cell Hybrid Clone Secreting Immunoglobulins of Both Parental Types. Nature. 1973;244:444–447. doi: 10.1038/244444a0. [ DOI ] [ PubMed ] [ Google Scholar ]
- 6. Köhler G., Milstein C. Continuous Cultures of Fused Cells Secreting Antibody of Predefined Specificity. Nature. 1975;256:495–497. doi: 10.1038/256495a0. [ DOI ] [ PubMed ] [ Google Scholar ]
- 7. Koprowski H., Steplewski Z., Herlyn D., Herlyn M. Study of Antibodies against Human Melanoma Produced by Somatic Cell Hybrids. Proc. Natl. Acad. Sci. USA. 1978;75:3405–3409. doi: 10.1073/pnas.75.7.3405. [ DOI ] [ PMC free article ] [ PubMed ] [ Google Scholar ]
- 8. Stashenko P., Antman K.H., Schlossman S.F. Serotherapy of a Patient with a Monoclonal Antibody Directed against a Human Lymphoma-Associated Antigen. Cancer Res. 1980;40:3147–3154. [ PubMed ] [ Google Scholar ]
- 9. Shin S.U., Morrison S.L. Production and Properties of Chimeric Antibody Molecules. Methods Enzymol. 1989;178:459–476. doi: 10.1016/0076-6879(89)78034-4. [ DOI ] [ PubMed ] [ Google Scholar ]
- 10. Riechmann L., Clark M., Waldmann H., Winter G. Reshaping Human Antibodies for Therapy. Nature. 1988;332:323–327. doi: 10.1038/332323a0. [ DOI ] [ PubMed ] [ Google Scholar ]
- 11. Nelson A.L., Dhimolea E., Reichert J.M. Development Trends for Human Monoclonal Antibody Therapeutics. Nat. Rev. Drug Discov. 2010;9:767–774. doi: 10.1038/nrd3229. [ DOI ] [ PubMed ] [ Google Scholar ]
- 12. Murphy K. Immunobiology. 9th ed. Garland Science; New York, NY, USA: 2017. [ DOI ] [ Google Scholar ]
- 13. Weiner L.M., Surana R., Wang S. Monoclonal Antibodies: Versatile Platforms for Cancer Immunotherapy. Nat. Rev. Immunol. 2010;10:317–327. doi: 10.1038/nri2744. [ DOI ] [ PMC free article ] [ PubMed ] [ Google Scholar ]
- 14. Li S., Schmitz K.R., Jeffrey P.D., Wiltzius J.J.W., Kussie P., Ferguson K.M. Structural Basis for Inhibition of the Epidermal Growth Factor Receptor by Cetuximab. Cancer Cell. 2005;7:301–311. doi: 10.1016/j.ccr.2005.03.003. [ DOI ] [ PubMed ] [ Google Scholar ]
- 15. Patel D., Bassi R., Hooper A., Prewett M., Hicklin D.J., Kang X. Anti-Epidermal Growth Factor Receptor Monoclonal Antibody Cetuximab Inhibits EGFR/HER-2 Heterodimerization and Activation. Int. J. Oncol. 2009;34:25–32. doi: 10.3892/ijo_00000125. [ DOI ] [ PubMed ] [ Google Scholar ]
- 16. Slamon D.J., Godolphin W., Jones L.A., Holt J.A., Wong S.G., Keith D.E., Levin W.J., Stuart S.G., Udove J., Ullrich A., et al. Studies of the HER-2/Neu Proto-Oncogene in Human Breast and Ovarian Cancer. Science. 1989;244:707–712. doi: 10.1126/science.2470152. [ DOI ] [ PubMed ] [ Google Scholar ]
- 17. Chen J.S., Lan K., Hung M.C. Strategies to Target HER2/Neu Overexpression for Cancer Therapy. Drug Resist. Updates. 2003;6:129–136. doi: 10.1016/S1368-7646(03)00040-2. [ DOI ] [ PubMed ] [ Google Scholar ]
- 18. Di Gaetano N., Cittera E., Nota R., Vecchi A., Grieco V., Scanziani E., Botto M., Introna M., Golay J. Complement Activation Determines the Therapeutic Activity of Rituximab in Vivo. J. Immunol. 2003;171:1581–1587. doi: 10.4049/jimmunol.171.3.1581. [ DOI ] [ PubMed ] [ Google Scholar ]
- 19. Racila E., Link B.K., Weng W.K., Witzig T.E., Ansell S., Maurer M.J., Huang J., Dahle C., Halwani A., Levy R., et al. A Polymorphism in the Complement Component C1qA Correlates with Prolonged Response Following Rituximab Therapy of Follicular Lymphoma. Clin. Cancer Res. 2008;14:6697–6703. doi: 10.1158/1078-0432.CCR-08-0745. [ DOI ] [ PMC free article ] [ PubMed ] [ Google Scholar ]
- 20. Coiffier B., Lepretre S., Pedersen L.M., Gadeberg O., Fredriksen H., Van Oers M.H.J., Wooldridge J., Kloczko J., Holowiecki J., Hellmann A., et al. Safety and Efficacy of Ofatumumab, a Fully Human Monoclonal Anti-CD20 Antibody, in Patients with Relapsed or Refractory B-Cell Chronic Lymphocytic Leukemia: A Phase 1-2 Study. Blood. 2008;111:1094–1100. doi: 10.1182/blood-2007-09-111781. [ DOI ] [ PubMed ] [ Google Scholar ]
- 21. Gül N., Babes L., Siegmund K., Korthouwer R., Bögels M., Braster R., Vidarsson G., Ten Hagen T.L.M., Kubes P., Van Egmond M. Macrophages Eliminate Circulating Tumor Cells after Monoclonal Antibody Therapy. J. Clin. Investig. 2014;124:812–823. doi: 10.1172/JCI66776. [ DOI ] [ PMC free article ] [ PubMed ] [ Google Scholar ]
- 22. Möller E. Contact-Induced Cytotoxicity by Lymphoid Cells Containing Foreign Isoantigens. Science. 1965;147:873–879. doi: 10.1126/science.147.3660.873. [ DOI ] [ PubMed ] [ Google Scholar ]
- 23. Teillaud J.-L. eLS. John Wiley & Sons, Ltd; Hoboken, NJ, USA: 2012. Antibody-Dependent Cellular Cytotoxicity (ADCC) [ DOI ] [ Google Scholar ]
- 24. Fanger M.W., Shen L., Graziano R.F., Guyre P.M. Cytotoxicity Mediated by Human Fc Receptors for IgG. Immunol. Today. 1989;10:92–99. doi: 10.1016/0167-5699(89)90234-X. [ DOI ] [ PubMed ] [ Google Scholar ]
- 25. Wallace P.K., Howell A.L., Fanger M.W. Role of Fcγ Receptors in Cancer and Infectious Disease. J. Leukoc. Biol. 1994;55:816–826. doi: 10.1002/jlb.55.6.816. [ DOI ] [ PubMed ] [ Google Scholar ]
- 26. Nimmerjahn F., Ravetch J.V. Fcγ Receptors as Regulators of Immune Responses. Nat. Rev. Immunol. 2008;8 doi: 10.1038/nri2206. [ DOI ] [ PubMed ] [ Google Scholar ]
- 27. De Saint Basile G., Ménasché G., Fischer A. Molecular Mechanisms of Biogenesis and Exocytosis of Cytotoxic Granules. Nat. Rev. Immunol. 2010;10:568–579. doi: 10.1038/nri2803. [ DOI ] [ PubMed ] [ Google Scholar ]
- 28. Nimmerjahn F., Ravetch J.V. Analyzing Antibody-Fc-Receptor Interactions. Methods Mol. Biol. 2008;415:151–162. doi: 10.1007/978-1-59745-570-1_9. [ DOI ] [ PubMed ] [ Google Scholar ]
- 29. Eischen C.M., Leibson P.J. Role for NK-Cell-Associated Fas Ligand in Cell-Mediated Cytotoxicity and Apoptosis. Res. Immunol. 1997;148:164–169. doi: 10.1016/S0923-2494(97)84219-8. [ DOI ] [ PubMed ] [ Google Scholar ]
- 30. Sondel P.M., Alderson K.L. Clinical Cancer Therapy by NK Cells via Antibody-Dependent Cell-Mediated Cytotoxicity. J. Biomed. Biotechnol. 2011;2011:379123. doi: 10.1155/2011/379123. [ DOI ] [ PMC free article ] [ PubMed ] [ Google Scholar ]
- 31. Clynes R.A., Towers T.L., Presta L.G., Ravetch J.V. Inhibitory Fc Receptors Modulate in Vivo Cytoxicity against Tumor Targets. Nat. Med. 2000;6:443–446. doi: 10.1038/74704. [ DOI ] [ PubMed ] [ Google Scholar ]
- 32. Minard-colin V., Xiu Y., Poe J.C., Horikawa M., Magro C.M., Hamaguchi Y., Haas K.M., Tedder T.F., Elisa C. Lymphoma Depletion during CD20 Immunotherapy in Mice Is Mediated By. Blood. 2008;112:1205–1213. doi: 10.1182/blood-2008-01-135160. [ DOI ] [ PMC free article ] [ PubMed ] [ Google Scholar ]
- 33. De Haij S., Jansen J.H.M., Boross P., Beurskens F.J., Bakema J.E., Bos D.L., Martens A., Verbeek J.S., Parren P.W.H.I., Van De Winkel J.G.J., et al. In Vivo Cytotoxicity of Type I CD20 Antibodies Critically Depends on Fc Receptor ITAM Signaling. Cancer Res. 2010;70:3209–3217. doi: 10.1158/0008-5472.CAN-09-4109. [ DOI ] [ PubMed ] [ Google Scholar ]
- 34. Hubert P., Heitzmann A., Viel S., Nicolas A., Sastre-Garau X., Oppezzo P., Pritsch O., Osinaga E., Amigorena S. Antibody-Dependent Cell Cytotoxicity Synapses Form in Mice during Tumor-Specific Antibody Immunotherapy. Cancer Res. 2011;71:5134–5143. doi: 10.1158/0008-5472.CAN-10-4222. [ DOI ] [ PubMed ] [ Google Scholar ]
- 35. Wu J., Edberg J.C., Redecha P.B., Bansal V., Guyre P.M., Coleman K., Salmon J.E., Kimberly R.P. A Novel Polymorphism of FcγRIIIa (CD16) Alters Receptor Function and Predisposes to Autoimmune Disease. J. Clin. Investig. 1997;100:1059–1070. doi: 10.1172/JCI119616. [ DOI ] [ PMC free article ] [ PubMed ] [ Google Scholar ]
- 36. Bibeau F., Lopez-Crapez E., Di Fiore F., Thezenas S., Ychou M., Blanchard F., Lamy A., Penault-Llorca F., Frébourg T., Michel P., et al. Impact of FcγRIIa-FcγRIIIa Polymorphisms and KRAS Mutations on the Clinical Outcome of Patients with Metastatic Colorectal Cancer Treated with Cetuximab plus Irinotecan. J. Clin. Oncol. 2009;27:1122–1129. doi: 10.1200/JCO.2008.18.0463. [ DOI ] [ PubMed ] [ Google Scholar ]
- 37. Cartron G., Dacheux L., Salles G., Solal-Celigny P., Bardos P., Colombat P., Watier H. Therapeutic Activity of Humanized Anti-CD20 Monoclonal Antibody and Polymorphism in IgG Fc Receptor FcγrIIIa Gene. Blood. 2002;99:754–758. doi: 10.1182/blood.V99.3.754. [ DOI ] [ PubMed ] [ Google Scholar ]
- 38. Weng W.K., Levy R. Two Immunoglobulin G Fragment C Receptor Polymorphisms Independently Predict Response to Rituximab in Patients with Follicular Lymphoma. J. Clin. Oncol. 2003;21:3940–3947. doi: 10.1200/JCO.2003.05.013. [ DOI ] [ PubMed ] [ Google Scholar ]
- 39. Hatjiharissi E., Xu L., Santos D.D., Hunter Z.R., Ciccarelli B.T., Verselis S., Modica M., Cao Y., Manning R.J., Leleu X., et al. Increased Natural Killer Cell Expression of CD16, Augmented Binding and ADCC Activity to Rituximab among Individuals Expressing the FcγRIIIa-158 v/v and V/F Polymorphism. Blood. 2007;110:2561–2564. doi: 10.1182/blood-2007-01-070656. [ DOI ] [ PMC free article ] [ PubMed ] [ Google Scholar ]
- 40. Rodríguez J., Zarate R., Bandres E., Boni V., Hernández A., Sola J.J., Honorato B., Bitarte N., García-Foncillas J. Fc Gamma Receptor Polymorphisms as Predictive Markers of Cetuximab Efficacy in Epidermal Growth Factor Receptor Downstream-Mutated Metastatic Colorectal Cancer. Eur. J. Cancer. 2012;48:1774–1780. doi: 10.1016/j.ejca.2012.01.007. [ DOI ] [ PubMed ] [ Google Scholar ]
- 41. Musolino A., Naldi N., Bortesi B., Pezzuolo D., Capelletti M., Missale G., Laccabue D., Zerbini A., Camisa R., Bisagni G., et al. Immunoglobulin g Fragment c Receptor Polymorphisms and Clinical Efficacy of Trastuzumab-Based Therapy in Patients with HER-2/Neu-Positive Metastatic Breast Cancer. J. Clin. Oncol. 2008;26:1789–1796. doi: 10.1200/JCO.2007.14.8957. [ DOI ] [ PubMed ] [ Google Scholar ]
- 42. Boero S., Morabito A., Banelli B., Cardinali B., Dozin B., Lunardi G., Piccioli P., Lastraioli S., Carosio R., Salvi S., et al. Analysis of in Vitro ADCC and Clinical Response to Trastuzumab: Possible Relevance of FcγRIIIA/FcγRIIA Gene Polymorphisms and HER-2 Expression Levels on Breast Cancer Cell Lines. J. Transl. Med. 2015;13:1–14. doi: 10.1186/s12967-015-0680-0. [ DOI ] [ PMC free article ] [ PubMed ] [ Google Scholar ]
- 43. Siebert N., Jensen C., Troschke-Meurer S., Zumpe M., Jüttner M., Ehlert K., Kietz S., Müller I., Lode H.N. Neuroblastoma Patients with High-Affinity FCGR2A, -3A and Stimulatory KIR 2DS2 Treated by Long-Term Infusion of Anti-GD2 Antibody Ch14.18/CHO Show Higher ADCC Levels and Improved Event-Free Survival. Oncoimmunology. 2016;5:1–14. doi: 10.1080/2162402X.2016.1235108. [ DOI ] [ PMC free article ] [ PubMed ] [ Google Scholar ]
- 44. Arnould L., Gelly M., Penault-Llorca F., Benoit L., Bonnetain F., Migeon C., Cabaret V., Fermeaux V., Bertheau P., Garnier J., et al. Trastuzumab-Based Treatment of HER2-Positive Breast Cancer: An Antibody-Dependent Cellular Cytotoxicity Mechanism? Br. J. Cancer. 2006;94:259–267. doi: 10.1038/sj.bjc.6602930. [ DOI ] [ PMC free article ] [ PubMed ] [ Google Scholar ]
- 45. Vermi W., Micheletti A., Finotti G., Tecchio C., Calzetti F., Costa S., Bugatti M., Calza S., Agostinelli C., Pileri S., et al. Slan + Monocytes and Macrophages Mediate CD20-Dependent B Cell Lymphoma Elimination via ADCC and ADCP. Cancer Res. 2018;78:3544–3559. doi: 10.1158/0008-5472.CAN-17-2344. [ DOI ] [ PubMed ] [ Google Scholar ]
- 46. de Weers M., Tai Y.-T., van der Veer M.S., Bakker J.M., Vink T., Jacobs D.C.H., Oomen L.A., Peipp M., Valerius T., Slootstra J.W., et al. Daratumumab, a Novel Therapeutic Human CD38 Monoclonal Antibody, Induces Killing of Multiple Myeloma and Other Hematological Tumors. J. Immunol. 2011;186:1840–1848. doi: 10.4049/jimmunol.1003032. [ DOI ] [ PubMed ] [ Google Scholar ]
- 47. Ferris R.L., Jaffee E.M., Ferrone S. Tumor Antigen-Targeted, Monoclonal Antibody-Based Immunotherapy: Clinical Response, Cellular Immunity, and Immunoescape. J. Clin. Oncol. 2010;28:4390–4399. doi: 10.1200/JCO.2009.27.6360. [ DOI ] [ PMC free article ] [ PubMed ] [ Google Scholar ]
- 48. Liu Z., Gunasekaran K., Wang W., Razinkov V., Sekirov L., Leng E., Sweet H., Foltz I., Howard M., Rousseau A.M., et al. Asymmetrical Fc Engineering Greatly Enhances Antibodydependent Cellular Cytotoxicity (ADCC) Effector Function and Stability of the Modified Antibodies. J. Biol. Chem. 2014;289:3571–3590. doi: 10.1074/jbc.M113.513366. [ DOI ] [ PMC free article ] [ PubMed ] [ Google Scholar ]
- 49. Umaña P., Jean-Mairet J., Moudry R., Amstutz H., Bailey J.E. Engineered Glycoforms of an Antineuroblastoma IgG1 with Optimized Antibody-Dependent Cellular Cytotoxic Activity. Nat. Biotechnol. 1999;17:176–180. doi: 10.1038/6179. [ DOI ] [ PubMed ] [ Google Scholar ]
- 50. Davies J., Jiang L., Pan L.Z., Labarre M.J., Anderson D., Reff M. Expression of GnTIII in a Recombinant Anti-CD20 CHO Production Cell Line: Expression of Antibodies with Altered Glycoforms Leads to an Increase in ADCC through Higher Affinity for FcγRIII. Biotechnol. Bioeng. 2001;74:288–294. doi: 10.1002/bit.1119. [ DOI ] [ PubMed ] [ Google Scholar ]
- 51. Shields R.L., Lai J., Keck R., O’Connell L.Y., Hong K., Gloria Meng Y., Weikert S.H.A., Presta L.G. Lack of Fucose on Human IgG1 N-Linked Oligosaccharide Improves Binding to Human FcγRIII and Antibody-Dependent Cellular Toxicity. J. Biol. Chem. 2002;277:26733–26740. doi: 10.1074/jbc.M202069200. [ DOI ] [ PubMed ] [ Google Scholar ]
- 52. Ishida T., Joh T., Uike N., Yamamoto K., Utsunomiya A., Yoshida S., Saburi Y., Miyamoto T., Takemoto S., Suzushima H., et al. Defucosylated Anti-CCR4 Monoclonal Antibody (KW-0761) for Relapsed Adult T-Cell Leukemia-Lymphoma: A Multicenter Phase II Study. J. Clin. Oncol. 2012;30:837–842. doi: 10.1200/JCO.2011.37.3472. [ DOI ] [ PubMed ] [ Google Scholar ]
- 53. Finn O.J. Human Tumor Antigens Yesterday, Today, and Tomorrow. Cancer Immunol. Res. 2017;5:347–354. doi: 10.1158/2326-6066.CIR-17-0112. [ DOI ] [ PMC free article ] [ PubMed ] [ Google Scholar ]
- 54. Maloney D.G., Grillo-López A.J., White C.A., Bodkin D., Schilder R.J., Neidhart J.A., Janakiraman N., Foon K.A., Liles T.M., Dallaire B.K., et al. IDEC-C2B8 (Rituximab) Anti-CD20 Monoclonal Antibody Therapy in Patients with Relapsed Low-Grade Non-Hodgkin’s Lymphoma. Blood. 1997;90:2188–2195. doi: 10.1182/blood.V90.6.2188. [ DOI ] [ PubMed ] [ Google Scholar ]
- 55. Rimawi M.F., Schiff R., Osborne C.K. Targeting HER2 for the Treatment of Breast Cancer. Annu. Rev. Med. 2015;66:111–128. doi: 10.1146/annurev-med-042513-015127. [ DOI ] [ PubMed ] [ Google Scholar ]
- 56. Mendelsohn J. The Epidermal Growth Factor Receptor as a Target for Therapy with Antireceptor Monoclonal Antibodies. Semin. Cancer Biol. 1990;1:339–344. [ PubMed ] [ Google Scholar ]
- 57. Indications and Year of First Approval for Each Antibody Were Accessed Using the FDA Drug Database. [(accessed on 21 May 2020)]; Available online: https://www.accessdata.fda.gov/scripts/cder/daf/
- 58. Chari R.V.J. Targeted Cancer Therapy: Conferring Specificity to Cytotoxic Drugs. Acc. Chem. Res. 2008;41:98–107. doi: 10.1021/ar700108g. [ DOI ] [ PubMed ] [ Google Scholar ]
- 59. Younes A., Bartlett N.L., Leonard J.P., Kennedy D.A., Lynch C.M., Sievers E.L., Forero-Torres A. Brentuximab Vedotin (SGN-35) for Relapsed CD30-Positive Lymphomas. N. Engl. J. Med. 2010;363:1812–1821. doi: 10.1056/NEJMoa1002965. [ DOI ] [ PubMed ] [ Google Scholar ]
- 60. Verma S., Miles D., Gianni L., Krop I.E., Welslau M., Baselga J., Pegram M., Oh D.Y., Diéras V., Guardino E., et al. Trastuzumab Emtansine for HER2-Positive Advanced Breast Cancer. N. Engl. J. Med. 2012;18:732–742. doi: 10.1056/NEJMoa1209124. [ DOI ] [ PMC free article ] [ PubMed ] [ Google Scholar ]
- 61. Chau C.H., Steeg P.S., Figg W.D. Antibody–Drug Conjugates for Cancer. Lancet. 2019;394:793–804. doi: 10.1016/S0140-6736(19)31774-X. [ DOI ] [ PubMed ] [ Google Scholar ]
- 62. Thomas A., Teicher B.A., Hassan R. Antibody–Drug Conjugates for Cancer Therapy. Lancet Oncol. 2016;17:e254–e262. doi: 10.1016/S1470-2045(16)30030-4. [ DOI ] [ PMC free article ] [ PubMed ] [ Google Scholar ]
- 63. Becker N., Benhar I. Antibody-Based Immunotoxins for the Treatment of Cancer. Antibodies. 2012;1:39–69. doi: 10.3390/antib1010039. [ DOI ] [ Google Scholar ]
- 64. Dhillon S. Moxetumomab Pasudotox: First Global Approval. Drugs. 2018;78:1763–1767. doi: 10.1007/s40265-018-1000-9. [ DOI ] [ PMC free article ] [ PubMed ] [ Google Scholar ]
- 65. Steiner M., Neri D. Antibody-Radionuclide Conjugates for Cancer Therapy: Historical Considerations and New Trends. Clin. Cancer Res. 2011;17:6406–6416. doi: 10.1158/1078-0432.CCR-11-0483. [ DOI ] [ PubMed ] [ Google Scholar ]
- 66. Ellis L.M., Hicklin D.J. VEGF-Targeted Therapy: Mechanisms of Anti-Tumour Activity. Nat. Rev. Cancer. 2008;8:579–591. doi: 10.1038/nrc2403. [ DOI ] [ PubMed ] [ Google Scholar ]
- 67. Krupitskaya Y., Wakelee H.A. Ramucirumab, a Fully Human MAb to the Transmembrane Signaling Tyrosine Kinase VEGFR-2 for the Potential Treatment of Cancer. Curr. Opin. Investig. Drugs. 2009;10:597–605. [ PubMed ] [ Google Scholar ]
- 68. Wu Y., Zhong Z., Huber J., Bassi R., Finnerty B., Corcoran E., Li H., Navarro E., Balderes P., Jimenez X., et al. Anti-Vascular Endothelial Growth Factor Receptor-1 Antagonist Antibody as a Therapeutic Agent for Cancer. Clin. Cancer Res. 2006;12:6573–6584. doi: 10.1158/1078-0432.CCR-06-0831. [ DOI ] [ PubMed ] [ Google Scholar ]
- 69. Shen J., Vil M.D., Prewett M., Damoci C., Zhang H., Li H., Jimenez X., Deevi D.S., Iacolina M., Kayas A., et al. Development of a Fully Human Anti-PDGFRβ Antibody That Suppresses Growth of Human Tumor Xenografts and Enhances Antitumor Activity of an Anti-VEGFR2 Antibody. Neoplasia. 2009;11:594–604. doi: 10.1593/neo.09278. [ DOI ] [ PMC free article ] [ PubMed ] [ Google Scholar ]
- 70. Colak S., ten Dijke P. Targeting TGF-β Signaling in Cancer. Trends Cancer. 2017;3:56–71. doi: 10.1016/j.trecan.2016.11.008. [ DOI ] [ PubMed ] [ Google Scholar ]
- 71. Grütter C., Wilkinson T., Turner R., Podichetty S., Finch D., McCourt M., Loning S., Jermutus L., Grütter M.G. A Cytokine-Neutralizing Antibody as a Structural Mimetic of 2 Receptor Interactions. Proc. Natl. Acad. Sci. USA. 2008;105:20251–20256. doi: 10.1073/pnas.0807200106. [ DOI ] [ PMC free article ] [ PubMed ] [ Google Scholar ]
- 72. Lutterbuese R., Raum T., Kischel R., Hoffmann P., Mangold S., Rattel B., Friedrich M., Thomas O., Lorenczewski G., Rau D., et al. T Cell-Engaging BiTE Antibodies Specific for EGFR Potently Eliminate KRAS- and BRAF-Mutated Colorectal Cancer Cells. Proc. Natl. Acad. Sci. USA. 2010;107:12605–12610. doi: 10.1073/pnas.1000976107. [ DOI ] [ PMC free article ] [ PubMed ] [ Google Scholar ]
- 73. Kantarjian H., Stein A., Gökbuget N., Fielding A.K., Schuh A.C., Ribera J.-M., Wei A., Dombret H., Foà R., Bassan R., et al. Blinatumomab versus Chemotherapy for Advanced Acute Lymphoblastic Leukemia. N. Engl. J. Med. 2017;376:836–847. doi: 10.1056/NEJMoa1609783. [ DOI ] [ PMC free article ] [ PubMed ] [ Google Scholar ]
- 74. Redman J.M., Hill E.M., AlDeghaither D., Weiner L.M. Mechanisms of Action of Therapeutic Antibodies for Cancer. Mol. Immunol. 2015;67:28–45. doi: 10.1016/j.molimm.2015.04.002. [ DOI ] [ PMC free article ] [ PubMed ] [ Google Scholar ]
- 75. Rech A.J., Vonderheide R.H. Clinical Use of Anti-CD25 Antibody Daclizumab to Enhance Immune Responses to Tumor Antigen Vaccination by Targeting Regulatory T Cells. Ann. N. Y. Acad. Sci. 2009;1174:99–106. doi: 10.1111/j.1749-6632.2009.04939.x. [ DOI ] [ PubMed ] [ Google Scholar ]
- 76. Pardoll D.M. The Blockade of Immune Checkpoints in Cancer Immunotherapy. Nat. Rev. Cancer. 2012 doi: 10.1038/nrc3239. [ DOI ] [ PMC free article ] [ PubMed ] [ Google Scholar ]
- 77. Qureshi O.S., Zheng Y., Nakamura K., Attridge K., Manzotti C., Schmidt E.M., Baker J., Jeffery L.E., Kaur S., Briggs Z., et al. Trans-Endocytosis of CD80 and CD86: A Molecular Basis for the Cell-Extrinsic Function of CTLA-4. Science. 2011;332:600–603. doi: 10.1126/science.1202947. [ DOI ] [ PMC free article ] [ PubMed ] [ Google Scholar ]
- 78. Leach D.R., Krummel M.F., Allison J.P. Enhancement of Antitumor Immunity by CTLA-4 Blockade. Science. 1996;271:1734–1736. doi: 10.1126/science.271.5256.1734. [ DOI ] [ PubMed ] [ Google Scholar ]
- 79. Hodi F.S., O’Day S.J., McDermott D.F., Weber R.W., Sosman J.A., Haanen J.B., Gonzalez R., Robert C., Schadendorf D., Hassel J.C., et al. Improved Survival with Ipilimumab in Patients with Metastatic Melanoma. N. Engl. J. Med. 2010;363:711–723. doi: 10.1056/NEJMoa1003466. [ DOI ] [ PMC free article ] [ PubMed ] [ Google Scholar ]
- 80. Topalian S.L., Drake C.G., Pardoll D.M. Immune Checkpoint Blockade: A Common Denominator Approach to Cancer Therapy. Cancer Cell. 2015;27:450–461. doi: 10.1016/j.ccell.2015.03.001. [ DOI ] [ PMC free article ] [ PubMed ] [ Google Scholar ]
- 81. Nishimura H., Nose M., Hiai H., Minato N., Honjo T. Development of Lupus-like Autoimmune Diseases by Disruption of the PD-1 Gene Encoding an ITIM Motif-Carrying Immunoreceptor. Immunity. 1999;11:141–151. doi: 10.1016/S1074-7613(00)80089-8. [ DOI ] [ PubMed ] [ Google Scholar ]
- 82. Topalian S.L., Hodi F.S., Brahmer J.R., Gettinger S.N., Smith D.C., McDermott D.F., Powderly J.D., Carvajal R.D., Sosman J.A., Atkins M.B., et al. Safety, Activity, and Immune Correlates of Anti-PD-1 Antibody in Cancer. N. Engl. J. Med. 2012;366:2443–2454. doi: 10.1056/NEJMoa1200690. [ DOI ] [ PMC free article ] [ PubMed ] [ Google Scholar ]
- 83. Sznol M., Chen L. Antagonist Antibodies to PD-1 and B7-H1 (PD-L1) in the Treatment of Advanced Human Cancer. Clin. Cancer Res. 2013;19:1021–1034. doi: 10.1158/1078-0432.CCR-12-2063. [ DOI ] [ PMC free article ] [ PubMed ] [ Google Scholar ]
- 84. Weber J.S., D’Angelo S.P., Minor D., Hodi F.S., Gutzmer R., Neyns B., Hoeller C., Khushalani N.I., Miller W.H., Lao C.D., et al. Nivolumab versus Chemotherapy in Patients with Advanced Melanoma Who Progressed after Anti-CTLA-4 Treatment (CheckMate 037): A Randomised, Controlled, Open-Label, Phase 3 Trial. Lancet Oncol. 2015;16:375–384. doi: 10.1016/S1470-2045(15)70076-8. [ DOI ] [ PubMed ] [ Google Scholar ]
- 85. Brahmer J., Reckamp K.L., Baas P., Crinò L., Eberhardt W.E.E., Poddubskaya E., Antonia S., Pluzanski A., Vokes E.E., Holgado E., et al. Nivolumab versus Docetaxel in Advanced Squamous-Cell Non-Small-Cell Lung Cancer. N. Engl. J. Med. 2015;373:123–135. doi: 10.1056/NEJMoa1504627. [ DOI ] [ PMC free article ] [ PubMed ] [ Google Scholar ]
- 86. Motzer R.J., Escudier B., McDermott D.F., George S., Hammers H.J., Srinivas S., Tykodi S.S., Sosman J.A., Procopio G., Plimack E.R., et al. Nivolumab versus Everolimus in Advanced Renal-Cell Carcinoma. N. Engl. J. Med. 2015;373:1803–1813. doi: 10.1056/NEJMoa1510665. [ DOI ] [ PMC free article ] [ PubMed ] [ Google Scholar ]
- 87. Ribas A., Puzanov I., Dummer R., Schadendorf D., Hamid O., Robert C., Hodi F.S., Schachter J., Pavlick A.C., Lewis K.D., et al. Pembrolizumab versus Investigator-Choice Chemotherapy for Ipilimumab-Refractory Melanoma (KEYNOTE-002): A Randomised, Controlled, Phase 2 Trial. Lancet Oncol. 2015;16:908–918. doi: 10.1016/S1470-2045(15)00083-2. [ DOI ] [ PMC free article ] [ PubMed ] [ Google Scholar ]
- 88. Abdin S.M., Zaher D.M., Arafa E.S.A., Omar H.A. Tackling Cancer Resistance by Immunotherapy: Updated Clinical Impact and Safety of PD-1/PD-L1 Inhibitors. Cancers. 2018;10:32. doi: 10.3390/cancers10020032. [ DOI ] [ PMC free article ] [ PubMed ] [ Google Scholar ]
- 89. Hargadon K.M., Johnson C.E., Williams C.J. Immune Checkpoint Blockade Therapy for Cancer: An Overview of FDA-Approved Immune Checkpoint Inhibitors. Int. Immunopharmacol. 2018;62:29–39. doi: 10.1016/j.intimp.2018.06.001. [ DOI ] [ PubMed ] [ Google Scholar ]
- 90. Anderson A.C., Joller N., Kuchroo V.K. Lag-3, Tim-3, and TIGIT: Co-Inhibitory Receptors with Specialized Functions in Immune Regulation. Immunity. 2016;44:989–1004. doi: 10.1016/j.immuni.2016.05.001. [ DOI ] [ PMC free article ] [ PubMed ] [ Google Scholar ]
- 91. Ni L., Dong C. New Checkpoints in Cancer Immunotherapy. Immunol. Rev. 2017;276:52–65. doi: 10.1111/imr.12524. [ DOI ] [ PubMed ] [ Google Scholar ]
- 92. Kim N., Kim H.S. Targeting Checkpoint Receptors and Molecules for Therapeutic Modulation of Natural Killer Cells. Front. Immunol. 2018;9:1–10. doi: 10.3389/fimmu.2018.02041. [ DOI ] [ PMC free article ] [ PubMed ] [ Google Scholar ]
- 93. Kohrt H.E., Thielens A., Marabelle A., Sagiv-Barfi I., Sola C., Chanuc F., Fuseri N., Bonnafous C., Czerwinski D., Rajapaksa A., et al. Anti-KIR Antibody Enhancement of Anti-Lymphoma Activity of Natural Killer Cells as Monotherapy and in Combination with Anti-CD20 Antibodies. Blood. 2014;123:678–686. doi: 10.1182/blood-2013-08-519199. [ DOI ] [ PMC free article ] [ PubMed ] [ Google Scholar ]
- 94. McLaughlin P., Grillo-López A.J., Link B.K., Levy R., Czuczman M.S., Williams M.E., Heyman M.R., Bence-Bruckler I., White C.A., Cabanillas F., et al. Rituximab Chimeric Anti-CD20 Monoclonal Antibody Therapy for Relapsed Indolent Lymphoma: Half of Patients Respond to a Four-Dose Treatment Program. J. Clin. Oncol. 1998;16:2825–2833. doi: 10.1200/JCO.1998.16.8.2825. [ DOI ] [ PubMed ] [ Google Scholar ]
- 95. Benavente S., Huang S., Armstrong E.A., Chi A., Hsu K.T., Wheeler D.L., Harari P.M. Establishment and Characterization of a Model of Acquired Resistance to Epidermal Growth Factor Receptor Targeting Agents in Human Cancer Cells. Clin. Cancer Res. 2009;15:1585–1592. doi: 10.1158/1078-0432.CCR-08-2068. [ DOI ] [ PMC free article ] [ PubMed ] [ Google Scholar ]
- 96. Ahmad A. Advances in Experimental Medicine and Biology. Volume 1152. Springer; Cham, Switzerland: 2019. Current Updates on Trastuzumab Resistance in HER2 Overexpressing Breast Cancers; pp. 217–228. [ DOI ] [ PubMed ] [ Google Scholar ]
- 97. Mishima Y., Terui Y., Takeuchi K., Matsumoto-Mishima Y., Matsusaka S., Utsubo-Kuniyoshi R., Hatake K. The Identification of Irreversible Rituximab-Resistant Lymphoma Caused by CD20 Gene Mutations. Blood Cancer J. 2011;1:e15–e18. doi: 10.1038/bcj.2011.11. [ DOI ] [ PMC free article ] [ PubMed ] [ Google Scholar ]
- 98. Sickmier E.A., Kurzeja R.J.M., Michelsen K., Vazir M., Yang E., Tasker A.S. The Panitumumab EGFR Complex Reveals a Binding Mechanism That Overcomes Cetuximab Induced Resistance. PLoS ONE. 2016;11:e0163366. doi: 10.1371/journal.pone.0163366. [ DOI ] [ PMC free article ] [ PubMed ] [ Google Scholar ]
- 99. Patel D., Lahiji A., Patel S., Franklin M., Jimenez X., Hicklin D.J., Kang X. Monoclonal Antibody Cetuximab Binds to and Down-Regulates Constitutively Activated Epidermal Growth Factor Receptor VIII on the Cell Surface. Anticancer Res. 2007;27:3355–3366. [ PubMed ] [ Google Scholar ]
- 100. Czuczman M.S., Olejniczak S., Gowda A., Kotowski A., Binder A., Kaur H., Knight J., Starostik P., Deans J., Hernandez-Ilizaliturri F.J. Acquirement of Rituximab Resistance in Lymphoma Cell Lines Is Associated with Both Global CD20 Gene and Protein Down-Regulation Regulated at the Pretranscriptional and Posttranscriptional Levels. Clin. Cancer Res. 2008;14:1561–1570. doi: 10.1158/1078-0432.CCR-07-1254. [ DOI ] [ PubMed ] [ Google Scholar ]
- 101. Nijhof I.S., Casneuf T., Van Velzen J., Van Kessel B., Axel A.E., Syed K., Groen R.W.J., Van Duin M., Sonneveld P., Minnema M.C., et al. CD38 Expression and Complement Inhibitors Affect Response and Resistance to Daratumumab Therapy in Myeloma. Blood. 2016;128:959–970. doi: 10.1182/blood-2016-03-703439. [ DOI ] [ PubMed ] [ Google Scholar ]
- 102. Seo Y., Ishii Y., Ochiai H., Fukuda K., Akimoto S., Hayashida T., Okabayashi K., Tsuruta M., Hasegawa H., Kitagawa Y. Cetuximab-Mediated ADCC Activity Is Correlated with the Cell Surface Expression Level of EGFR but Not with the KRAS/BRAF Mutational Status in Colorectal Cancer. Oncol. Rep. 2014;31:2115–2122. doi: 10.3892/or.2014.3077. [ DOI ] [ PubMed ] [ Google Scholar ]
- 103. Lee S.C., López-Albaitero A., Ferris R.L. Immunotherapy of Head and Neck Cancer Using Tumor Antigen-Specific Monoclonal Antibodies. Curr. Oncol. Rep. 2009;11:156–162. doi: 10.1007/s11912-009-0023-5. [ DOI ] [ PubMed ] [ Google Scholar ]
- 104. Braig F., Kriegs M., Voigtlaender M., Habel B., Grob T., Biskup K., Blanchard V., Sack M., Thalhammer A., Batalla I.B., et al. Cetuximab Resistance in Head and Neck Cancer Is Mediated by EGFR-K521 Polymorphism. Cancer Res. 2017;77:1188–1199. doi: 10.1158/0008-5472.CAN-16-0754. [ DOI ] [ PubMed ] [ Google Scholar ]
- 105. Nakadate Y., Kodera Y., Kitamura Y., Shirasawa S., Tachibana T., Tamura T., Koizumi F. KRAS Mutation Confers Resistance to Antibody-Dependent Cellular Cytotoxicity of Cetuximab against Human Colorectal Cancer Cells. Int. J. Cancer. 2014;134:2146–2155. doi: 10.1002/ijc.28550. [ DOI ] [ PubMed ] [ Google Scholar ]
- 106. Valabrega G., Montemurro F., Aglietta M. Trastuzumab: Mechanism of Action, Resistance and Future Perspectives in HER2-Overexpressing Breast Cancer. Ann. Oncol. 2007;18:977–984. doi: 10.1093/annonc/mdl475. [ DOI ] [ PubMed ] [ Google Scholar ]
- 107. Gennari R., Menard S., Fagnoni F., Ponchio L., Scelsi M., Tagliabue E., Castiglioni F., Villani L., Magalotti C., Gibelli N., et al. Pilot Study of the Mechanism of Action of Preoperative Trastuzumab in Patients with Primary Operable Breast Tumors Overexpressing HER2. Clin. Cancer Res. 2004;10:5650–5655. doi: 10.1158/1078-0432.CCR-04-0225. [ DOI ] [ PubMed ] [ Google Scholar ]
- 108. Kominsky S.L., Hobeika A.C., Lake F.A., Torres B.A., Johnson H.M. Down-Regulation of Neu/HER-2 by Interferon-γ in Prostate Cancer Cells. Cancer Res. 2000;60:3904–3908. [ PubMed ] [ Google Scholar ]
- 109. Shi Y., Fan X., Meng W., Deng H., Zhang N., An Z. Engagement of Immune Effector Cells by Trastuzumab Induces HER2/ERBB2 Downregulation in Cancer Cells through STAT1 Activation. Breast Cancer Res. 2014;16:1–11. doi: 10.1186/bcr3637. [ DOI ] [ PMC free article ] [ PubMed ] [ Google Scholar ]
- 110. Bellucci R., Martin A., Bommarito D., Wang K., Hansen S.H., Freeman G.J., Ritz J. Interferon-γ-Induced Activation of JAK1 and JAK2 Suppresses Tumor Cell Susceptibility to NK Cells through Upregulation of PD-L1 Expression. Oncoimmunology. 2015;4:1–10. doi: 10.1080/2162402X.2015.1008824. [ DOI ] [ PMC free article ] [ PubMed ] [ Google Scholar ]
- 111. Sforza V., Martinelli E., Ciardiello F., Gambardella V., Napolitano S., Martini G., Corte C.D., Cardone C., Ferrara M.L., Reginelli A., et al. Mechanisms of Resistance to Anti-Epidermal Growth Factor Receptor Inhibitors in Metastatic Colorectal Cancer. World J. Gastroenterol. 2016;22:6345–6361. doi: 10.3748/wjg.v22.i28.6345. [ DOI ] [ PMC free article ] [ PubMed ] [ Google Scholar ]
- 112. Misale S., Yaeger R., Hobor S., Scala E., Janakiraman M., Liska D., Valtorta E., Schiavo R., Buscarino M., Siravegna G., et al. Emergence of KRAS Mutations and Acquired Resistance to Anti-EGFR Therapy in Colorectal Cancer. Nature. 2012;486:532–536. doi: 10.1038/nature11156. [ DOI ] [ PMC free article ] [ PubMed ] [ Google Scholar ]
- 113. Kasper S., Reis H., Ziegler S., Nothdurft S., Mueller A., Goetz M., Wiesweg M., Phasue J., Ting S., Wieczorek S., et al. Molecular Dissection of Effector Mechanisms of RAS-Mediated Resistance to Anti-EGFR Antibody Therapy. Oncotarget. 2017;8:45898–45917. doi: 10.18632/oncotarget.17438. [ DOI ] [ PMC free article ] [ PubMed ] [ Google Scholar ]
- 114. Cuyàs E., Queralt B., Martin-Castillo B., Bosch-Barrera J., Menendez J.A. EphA2 Receptor Activation with Ephrin-A1 Ligand Restores Cetuximab Efficacy in NRAS-Mutant Colorectal Cancer Cells. Oncol. Rep. 2017;38:263–270. doi: 10.3892/or.2017.5682. [ DOI ] [ PubMed ] [ Google Scholar ]
- 115. Ozawa H., Ranaweera R.S., Izumchenko E., Makarev E., Zhavoronkov A., Fertig E.J., Howard J.D., Markovic A., Bedi A., Ravi R., et al. SMAD4 Loss Is Associated with Cetuximab Resistance and Induction of MAPK/JNK Activation in Head and Neck Cancer Cells. Clin. Cancer Res. 2017;23:5162–5175. doi: 10.1158/1078-0432.CCR-16-1686. [ DOI ] [ PMC free article ] [ PubMed ] [ Google Scholar ]
- 116. Xue F., Liu Y., Chu H., Wen Y., Yan L., Tang Q., Xiao E., Zhang D., Zhang H. EIF5A2 Is an Alternative Pathway for Cell Proliferation in Cetuximab-Treated Epithelial Hepatocellular Carcinoma. Am. J. Transl. Res. 2016;8:4670–4681. [ PMC free article ] [ PubMed ] [ Google Scholar ]
- 117. Kong X., Zhang K., Wang X., Yang X., Li Y., Zhai J., Xing Z., Qi Y., Gao R., Feng X., et al. Mechanism of Trastuzumab Resistance Caused by HER-2 Mutation in Breast Carcinomas. Cancer Manag. Res. 2019;11:5971–5982. doi: 10.2147/CMAR.S194137. [ DOI ] [ PMC free article ] [ PubMed ] [ Google Scholar ]
- 118. Chandarlapaty S., Sakr R.A., Giri D., Patil S., Heguy A., Morrow M., Modi S., Norton L., Rosen N., Hudis C., et al. Frequent Mutational Activation of the PI3K-AKT Pathway in Trastuzumab-Resistant Breast Cancer. Clin. Cancer Res. 2012;18:6784–6791. doi: 10.1158/1078-0432.CCR-12-1785. [ DOI ] [ PMC free article ] [ PubMed ] [ Google Scholar ]
- 119. Ritter C.A., Perez-Torres M., Rinehart C., Guix M., Dugger T., Engelman J.A., Arteaga C.L. Human Breast Cancer Cells Selected for Resistance to Trastuzumab in Vivo Overexpress Epidermal Growth Factor Receptor and ErbB Ligands and Remain Dependent on the ErbB Receptor Network. Clin. Cancer Res. 2007;13:4909–4919. doi: 10.1158/1078-0432.CCR-07-0701. [ DOI ] [ PubMed ] [ Google Scholar ]
- 120. Vernieri C., Milano M., Brambilla M., Mennitto A., Maggi C., Cona M.S., Prisciandaro M., Fabbroni C., Celio L., Mariani G., et al. Resistance Mechanisms to Anti-HER2 Therapies in HER2-Positive Breast Cancer: Current Knowledge, New Research Directions and Therapeutic Perspectives. Crit. Rev. Oncol. Hematol. 2019;139:53–66. doi: 10.1016/j.critrevonc.2019.05.001. [ DOI ] [ PubMed ] [ Google Scholar ]
- 121. Kawaguchi Y., Kono K., Mizukami Y., Mimura K., Fujii H. Mechanisms of Escape from Trastuzumab-Mediated ADCC in Esophageal Squamous Cell Carcinoma: Relation to Susceptibility to Perforin-Granzyme. Anticancer Res. 2009;29:2137–2146. [ PubMed ] [ Google Scholar ]
- 122. Evans M.K., Sauer S.J., Nath S., Robinson T.J., Morse M.A., Devi G.R. X-Linked Inhibitor of Apoptosis Protein Mediates Tumor Cell Resistance to Antibody-Dependent Cellular Cytotoxicity. Cell Death Dis. 2016;7:e2073. doi: 10.1038/cddis.2015.412. [ DOI ] [ PMC free article ] [ PubMed ] [ Google Scholar ]
- 123. Jazirehi A.R., Vega M.I., Bonavida B. Development of Rituximab-Resistant Lymphoma Clones with Altered Cell Signaling and Cross-Resistance to Chemotherapy. Cancer Res. 2007;67:1270–1281. doi: 10.1158/0008-5472.CAN-06-2184. [ DOI ] [ PubMed ] [ Google Scholar ]
- 124. Schmitz S., Bindea G., Albu R.I., Mlecnik B., Machiels J.P. Cetuximab Promotes Epithelial to Mesenchymal Transition and Cancer Associated Fibroblasts in Patients with Head and Neck Cancer. Oncotarget. 2015;6:34288–34299. doi: 10.18632/oncotarget.5924. [ DOI ] [ PMC free article ] [ PubMed ] [ Google Scholar ]
- 125. Kimura I., Kitahara H., Ooi K., Kato K., Noguchi N., Yoshizawa K., Nakamura H., Kawashiri S. Loss of Epidermal Growth Factor Receptor Expression in Oral Squamous Cell Carcinoma Is Associated with Invasiveness and Epithelial-Mesenchymal Transition. Oncol. Lett. 2016;11:201–207. doi: 10.3892/ol.2015.3833. [ DOI ] [ PMC free article ] [ PubMed ] [ Google Scholar ]
- 126. Hsu D.S.S., Hwang W.L., Yuh C.H., Chu C.H., Ho Y.H., Chen P.B., Lin H.S., Lin H.K., Wu S.P., Lin C.Y., et al. Lymphotoxin-β Interacts with Methylated EGFR to Mediate Acquired Resistance to Cetuximab in Head and Neck Cancer. Clin. Cancer Res. 2017;23:4388–4401. doi: 10.1158/1078-0432.CCR-16-1955. [ DOI ] [ PubMed ] [ Google Scholar ]
- 127. Cheng H., Fertig E.J., Ozawa H., Hatakeyama H., Howard J.D., Perez J., Considine M., Thakar M., Ranaweera R., Krigsfeld G., et al. Decreased SMAD4 Expression Is Associated with Induction of Epithelial-to-Mesenchymal Transition and Cetuximab Resistance in Head and Neck Squamous Cell Carcinoma. Cancer Biol. Ther. 2015;16:1252–1258. doi: 10.1080/15384047.2015.1056418. [ DOI ] [ PMC free article ] [ PubMed ] [ Google Scholar ]
- 128. Oliveras-Ferraros C., Vazquez-Martin A., Cufí S., Queralt B., Báez L., Guardeño R., Hernández-Yagü e X., Martin-Castillo B., Brunet J., Menendez J.A. Stem Cell Property Epithelial-to-Mesenchymal Transition Is a Core Transcriptional Network for Predicting Cetuximab (ErbituxTM) Efficacy in KRAS Wild-Type Tumor Cells. J. Cell. Biochem. 2011;112:10–29. doi: 10.1002/jcb.22952. [ DOI ] [ PubMed ] [ Google Scholar ]
- 129. Oliveras-Ferraros C., Corominas-Faja B., Vazquez-Martin S.C.A., Martin-Castillo B., Iglesias J.M., López-Bonet E., Martin Á.G., Menendez J.A. Epithelial-to-Mesenchymal Transition (EMT) Confers Primary Resistance to Trastuzumab (Herceptin) Cell Cycle. 2012;11:4020–4032. doi: 10.4161/cc.22225. [ DOI ] [ PMC free article ] [ PubMed ] [ Google Scholar ]
- 130. Burnett J.P., Korkaya H., Ouzounova M.D., Jiang H., Conley S.J., Newman B.W., Sun L., Connarn J.N., Chen C.S., Zhang N., et al. Trastuzumab Resistance Induces EMT to Transform HER2 + PTEN’ to a Triple Negative Breast Cancer That Requires Unique Treatment Options. Sci. Rep. 2015;5:15821. doi: 10.1038/srep15821. [ DOI ] [ PMC free article ] [ PubMed ] [ Google Scholar ]
- 131. Capuano C., Romanelli M., Pighi C., Cimino G., Rago A., Molfetta R., Paolini R., Santoni A., Galandrini R. Anti-CD20 Therapy Acts via FcγRIIIA to Diminish Responsiveness of Human Natural Killer Cells. Cancer Res. 2015;75:4097–4108. doi: 10.1158/0008-5472.CAN-15-0781. [ DOI ] [ PubMed ] [ Google Scholar ]
- 132. Xu F., Sunderland A., Zhou Y., Schulick R.D., Edil B.H., Zhu Y. Blockade of CD112R and TIGIT Signaling Sensitizes Human Natural Killer Cell Functions. Cancer Immunol. Immunother. 2017;66:1367–1375. doi: 10.1007/s00262-017-2031-x. [ DOI ] [ PMC free article ] [ PubMed ] [ Google Scholar ]
- 133. Cooley S., Burns L.J., Repka T., Miller J.S. Natural Killer Cell Cytotoxicity of Breast Cancer Targets Is Enhanced by Two Distinct Mechanisms of Antibody-Dependent Cellular Cytotoxicity against LFA-3 and HER2/Neu. Exp. Hematol. 1999;27:1533–1541. doi: 10.1016/S0301-472X(99)00089-2. [ DOI ] [ PubMed ] [ Google Scholar ]
- 134. Sordo-Bahamonde C., Vitale M., Lorenzo-Herrero S., López-Soto A., Gonzalez S. Mechanisms of Resistance to NK Cell Immunotherapy. Cancers. 2020;12:893. doi: 10.3390/cancers12040893. [ DOI ] [ PMC free article ] [ PubMed ] [ Google Scholar ]
- 135. Aldeghaither D.S., Zahavi D.J., Murray J.C., Fertig E.J., Graham G.T., Zhang Y.-W., O’Connell A., Ma J., Jablonski S.A., Weiner L.M. A Mechanism of Resistance to Antibody-Targeted Immune Attack. Cancer Immunol. Res. 2019;7:230–243. doi: 10.1158/2326-6066.CIR-18-0266. [ DOI ] [ PMC free article ] [ PubMed ] [ Google Scholar ]
- 136. Corraliza-Gorjón I., Somovilla-Crespo B., Santamaria S., Garcia-Sanz J.A., Kremer L. New Strategies Using Antibody Combinations to Increase Cancer Treatment Effectiveness. Front. Immunol. 2017;8:1804. doi: 10.3389/fimmu.2017.01804. [ DOI ] [ PMC free article ] [ PubMed ] [ Google Scholar ]
- 137. Linares J., Rullan A., Taberna M., Vazquez S., Mesia R. Emergence of Long-Term Surviving Patients with the Introduction of Cetuximab in Recurrent/Metastatic Disease of Squamous Cell Carcinoma of Head and Neck. Oral Oncol. 2016;100:e4. doi: 10.1016/j.oraloncology.2016.02.006. [ DOI ] [ PubMed ] [ Google Scholar ]
- 138. Melero I., Berman D.M., Aznar M.A., Korman A.J., Gracia J.L.P., Haanen J. Evolving Synergistic Combinations of Targeted Immunotherapies to Combat Cancer. Nat. Rev. Cancer. 2015;15:457–472. doi: 10.1038/nrc3973. [ DOI ] [ PubMed ] [ Google Scholar ]
- 139. Stagg J., Loi S., Divisekera U., Ngiow S.F., Duret H., Yagita H., Teng M.W., Smyth M.J. Anti-ErbB-2 MAb Therapy Requires Type I and II Interferons and Synergizes with Anti-PD-1 or Anti-CD137 MAb Therapy. Proc. Natl. Acad. Sci. USA. 2011;108:7142–7147. doi: 10.1073/pnas.1016569108. [ DOI ] [ PMC free article ] [ PubMed ] [ Google Scholar ]
- 140. Griguolo G., Pascual T., Dieci M.V., Guarneri V., Prat A. Interaction of Host Immunity with HER2-Targeted Treatment and Tumor Heterogeneity in HER2-Positive Breast Cancer. J. Immunother. Cancer. 2019;7:90. doi: 10.1186/s40425-019-0548-6. [ DOI ] [ PMC free article ] [ PubMed ] [ Google Scholar ]
- 141. Loi S., Giobbie-Hurder A., Gombos A., Bachelot T., Hui R., Curigliano G., Campone M., Biganzoli L., Bonnefoi H., Jerusalem G., et al. Pembrolizumab plus Trastuzumab in Trastuzumab-Resistant, Advanced, HER2-Positive Breast Cancer (PANACEA): A Single-Arm, Multicentre, Phase 1b–2 Trial. Lancet Oncol. 2019;20:371–382. doi: 10.1016/S1470-2045(18)30812-X. [ DOI ] [ PubMed ] [ Google Scholar ]
- 142. Korman A., Chen B., Wang C., Wu L., Cardarelli P., Selby M. Activity of Anti-PD-1 in Murine Tumor Models: Role of “Host” PD-L1 and Synergistic Effect of Anti-PD-1 and Anti-CTLA-4 (48.37) J. Immunol. 2007;178(Suppl. 1):S82. [ Google Scholar ]
- 143. Larkin J., Chiarion-Sileni V., Gonzalez R., Grob J.J., Cowey C.L., Lao C.D., Schadendorf D., Dummer R., Smylie M., Rutkowski P., et al. Combined Nivolumab and Ipilimumab or Monotherapy in Untreated Melanoma. N. Engl. J. Med. 2015;373:23–34. doi: 10.1056/NEJMoa1504030. [ DOI ] [ PMC free article ] [ PubMed ] [ Google Scholar ]
- 144. Siu L.L., Steeghs N., Meniawy T., Joerger M., Spratlin J.L., Rottey S., Nagrial A., Cooper A., Meier R., Guan X., et al. Preliminary Results of a Phase I/IIa Study of BMS-986156 (Glucocorticoid-Induced Tumor Necrosis Factor Receptor–Related Gene (GITR) Agonist), Alone and in Combination with Nivolumab in Pts with Advanced Solid Tumors. J. Clin. Oncol. 2017 doi: 10.1200/JCO.2017.35.15_suppl.104. [ DOI ] [ Google Scholar ]
- View on publisher site
- PDF (1.1 MB)
- Collections
Similar articles
Cited by other articles, links to ncbi databases.
- Download .nbib .nbib
- Format: AMA APA MLA NLM
Add to Collections

IMAGES